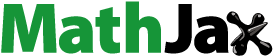
Abstract
Utilizing a non-Newtonian lubricant, such as Bingham plastic fluid, in a hydrodynamic journal bearing affords the opportunity to actively alter the operating performance of the bearing. In this work, the main purpose is to explore the behavior of the journal bearing lubricated by Bingham plastic using the computational fluid dynamics (CFD) method considering roughness and cavitation. Here, the concept of an engineered partial roughness pattern is proposed to create positive effects in improving tribological and acoustic performances under different roughness levels and eccentricity ratios. The cavitation phenomena is modeled using the mixture multiphase cavitation approach to get a more realistic result. The numerical results of this study indicate that the surface roughness level of the roughed journal bearing has a significant effect on the load-carrying capacity irrespective of the eccentricity ratio. Moreover, the larger the roughness level, the lower the load-carrying capacity. It is also found that compared to bearings without roughness, it is possible to achieve a 5% and 2% reduction in noise level and friction force, respectively, when the ”rough” level is employed on the roughed bearing. The findings of this research show that artificial partial roughness may be useful for enhancing journal bearing indices.
Public Interest Statement
In recent years, it has been demonstrated that the introduction of surface roughness can alter the bearing performance. In this study, the beneficial effect of the roughed surface of the bearing on tribological and noise performance is numerically examined. The numerical result indicates that introducing the roughness can reduce the friction and noise level of the bearing. The results of this study can be used as a theoretical foundation for obtaining high-performance journal bearings lubricated by a non-Newtonian fluid.
1. Introduction
The relative motion of two engine components is of great concern because it affects the long-term performance of the mechanical system. Bearing systems are the devices utilized to support the various moving machine components due to their simplicity, and a high degree of precision. Radially loaded hydrodynamic journal bearings are one of the most widely utilized industrial bearings. In general, journal bearings are widely used in turbines, compressors, and pumps. Journal bearings are utilized due to their capacity to withstand heavy loads and high speeds safely and steadily (Chen & Zhang, Citation2019).
In a real application, lubrication in journal bearings has a very important role. The lubrication mechanism must be engineered to function under varying loads, speeds, and conditions with low energy consumption, good performance, and minimum noise (Marey et al., Citation2021). Consequently, a number of studies have concentrated on lubricant characteristics that have a substantial effect on the functional operation of bearings. Currently, non-Newtonian fluids and their effect on the characteristics of lubricated contacts are of primary importance. This is because of the anti-wear, low-friction, and high-pressure characteristics inherent to non-Newtonian fluids (Dang et al., Citation2020). The properties of shear stress and shear strain in Newtonian fluids have linear properties, while in non-Newtonian fluids they are nonlinear. Gertzos et al. (Citation2008) investigated journal bearings lubricated with Bingham fluid, through three-dimensional analysis with CFD, using FLUENT software. The authors provided the designer engineer with the Raimondi and Boyd charts that they might utilize to create smart journal bearings. Lin et al. (Citation2016) investigated journal bearings lubricated by Newtonian fluids mixed with some modified substances. Several properties as dilation and pseudo-plasticity have been analyzed. The authors conclude that the load-bearing capacity and friction loss are relatively higher in the case of non-Newtonian lubricants. However, the opposite conclusion was noted in the case of pseudo-plastic fluids. Javorova et al. (Citation2016a) investigated the operation of a journal bearing with a finite length while considering the effects of non-Newtonian Rabinowitsch flow rheology. They came to the conclusion that, as compared to Newtonian lubricants, dilatant lubricants had higher values of film pressure and load support, but the situation was the opposite for pseudoplastic lubricants. Vaz et al. (Citation2017) discussed the viability of utilizing magnetorheological (MR) fluid as a lubricant. They demonstrated that the addition of MR fluid could enhance the load-carrying capacity of the journal bearing. In the work of Lampaert and van Ostayen (Citation2019, Citation2020), an accurate analytical model for hydrostatic bearings using Bingham plastic fluid was obtained. The model showed the potential use of magnetorheological or electrorheological fluids in such a way that yield stress can be controlled. Moreover, Dang et al. (Citation2020) confirmed that non-Newtonian lubricants perform better than Newtonian lubricants in journal bearings under specific operating circumstances. Therefore, since viscosity affects the hydrodynamic characteristics of lubricated bearings, the influence of non-Newtonian lubricant behavior cannot be ignored, as discussed by Kouidera et al. (Citation2021). In recent publication, in the case of hydrophobic journal bearings, it was also demonstrated that non-Newtonian lubricant has a more significant effect on reducing noise than Newtonian lubricant (Tauviqirrahman et al., Citation2022). However, relatively little research has been published in the literature that emphasizes the properties of bearings with Bingham plastic while taking roughness into account.
In the bearing manufacturing processes, there is a manufacturing process that uses grinding (Stout & Rowe, Citation1974). Grinding is used to finish workpieces that require a high level of surface quality as well as shape and dimension precision. This process affects the level of roughness on the outer and inner surfaces of the bearing. The level of surface roughness has a very large effect on the characteristics of the bearing. Bhaskar et al. (Citation2013) investigated the characteristics of bearings with three distinct orientations of surface roughness. They revealed that the load-supporting capacity of a bearing with longitudinal surface roughness is 52.57% greater than that of an ideal smooth bearing with zero surface roughness. A similar result was observed by Hsu et al. (Citation2013). Meanwhile, when longitudinal roughness was used in the case of curvilinear squeeze film bearing, the opposite result was seen, as discussed by Walicka et al. (Citation2016). Compared to the case of smooth bearing surfaces, they noticed that longitudinal roughness provokes slight decreases in pressure values, whereas circumferential roughness induces pressure increases. Later, Javorova et al. (Citation2016b) found that when compared to the performance of bearings with smooth surfaces and Newtonian lubricant, there was a decrease in the values of maximum pressure and load-carrying capacity for the journal bearing with transverse roughness and pseudo-plastic. Kalavathi et al. (Citation2016), based on the Reynolds approach, also noticed that the load-bearing capacity increases with surface roughness. Cui et al. (Citation2018) investigated how surface roughness affected the bearing transient behavior during the start-up period. They showed that the longitudinal roughness arrangement significantly affects the hydrodynamic force reduction. Abd Al-Samieh (Citation2019) concluded that raising the amplitude of surface waviness produces both an increase in pressure fluctuations and a decrease in film thickness.
Based on the research of Frene et al. (Citation2006), journal bearing with very small clearance has a flow that is not always laminar. The flow will become entirely turbulent when the real Reynolds number exceeds the critical Reynolds number. When the flow reaches turbulent flow, according to Proudman (Citation1952), it will move arbitrarily. Thus, he indicated that turbulent flow can produce noise. It is noted that some recently published articles investigated the acoustic performance of journal bearings under turbulent conditions using the computational fluid dynamics (CFD) method. These works indicated that modifying the surface such as texturing/roughening (F. M. Meng & Zhang, Citation2018; F. Meng et al., Citation2020; Tauviqirrahman, Jamari, et al., Citation2021) or coating (Tauviqirrahman, Afif, et al., Citation2021) the surface could significantly affect the bearing’s noise level. All of these papers, however, focused on the Newtonian fluid as a lubricant. References on the acoustic indices of journal bearings with a non-Newtonian lubricant are still lacking.
This study aims to analyze the performance of journal bearings with non-Newtonian Bingham plastic fluid both tribological and acoustic behavior considering the surface roughness. Here, the bearing surface is partially roughed with a specified roughness level, while the other has a smooth surface. Various eccentricity ratios of the bearing are evaluated for the bearing. Other factors are also taken into account to ensure that the conditions simulated are as realistic as possible. Consider multiphase cavitation for example. Due to the mass flow-conserving characteristic, the rupture and reformation boundaries during cavitation are defined in this way without the need for additional boundary conditions. Using an improved model, namely vapor-liquid two-phase flow, it is feasible to accurately predict the acoustic behavior and vapor volume fraction.
2. Materials and methods
2.1 Governing equations
In this study, we solve the flow behavior caused by surface motion by computing the Navier-Stokes for incompressible flow. For this investigation, the Reynolds-averaged Navier—Stokes (RANS) equation in conjunction with the equation for the conservation of mass is utilized (ANSYS, Citation2019).
The RANS equation reads:
The conservation of mass (continuity) equation reads:
The bearing noise is of special significance in this acoustic investigation. Because of the turbulence in the lubricant, the noise seems to develop during bearing operation. A computational technique to find solutions to the noise generated in the lubricant is used in this study, which employs the broadband noise source model (ANSYS, Citation2019). The acoustic power level PA is stated here:
The rescaled constant has been given the value of 0.1 here.
2.2 Bingham plastic
Bingham plastic lubricants are non-Newtonian fluids that have a linear stress-strain relationship and require a finite yield stress prior to beginning to flow. In this study, Bingham plastic is approached using Herschel—Bulkley model. It reads (ANSYS, Inc, Citation2019):
where μ is non-Newtonian viscosity (Pa.s), is shear rate (s−1),
is yield stress (Pa),
is yield viscosity (Pa.s), ki is consistency index (kg sn-2/m) and n is the dimensionless power-law index. It should be noted that by setting k = μ, n = 1 and
, the characteristic of Bingham plastic can be obtained for simulation. Here, the yield viscosity and yield stress are set to 500 Pa.s and 213.8 Pa, respectively.
2.3 Surface roughness modelling
In the present work, a sand grain model, as depicted in Figure , is used to simulate the effect of a real, rough surface on lubricant flow within the bearing. In this instance, the surface is uniformly covered by a monolayer of closely packed spheres of a given diameter Ks. The roughness height Ks represents the equivalent sand grain roughness height, which differs from the geometric surface roughness height. The height of the surface geometric roughness must be converted to an equivalent sand grain roughness using a conversion factor. In this study, Ra is used as the parameter to indicate roughness height Ks. Ra represents the arithmetic mean of the roughness pattern and is in fact measured with a profilometer. For all calculations here, the value of Ra will be the input to determine the roughness of the bearing. According to the experiment conducted by Adams et al. (Citation2012), the correlation between Ks and Ra can be defined as follows:
Figure 1. Schematic of uniform sand-grain roughness model (Adams et al., Citation2012; ANSYS, Citation2019).

The roughness level is introduced in this work to indicate the value of Ra of the partially roughed journal bearing. The roughness level is classified as ideal, fine, medium, or rough for all subsequent simulations, as detailed in Table .
Table 1. Surface roughness level (JIS, Citation2013)
2.4 Cavitation model
The vapor transport equation governs the liquid-vapor mass transfer (evaporation and condensation) in cavitation. It reads:
A model provided by Zwart-Gerber-Belamri (Zwart et al., Citation2004) is employed to account for the cavitation effect, in which all bubbles are taken to be the same size in the system. Zwart-Gerber-Belamri provided the following formulations based on generalized Rayleigh-Plesset equations to account for the phase change rates during vapor formation and condensation (Zwart et al., Citation2004).
where pv denotes the vapor pressure, Fv refers to the coefficient of evaporation, and Fcond is the coefficient of condensation.
3. Materials and methods
3.1 Geometry
The basic geometry of the journal bearing utilized to simulate various cases adopts the main geometry of the journal bearing as presented in (Gertzos et al., Citation2008). In the present study, a rough surface with many levels is applied to the leading edge of the contact, while the rest of the contact area is assumed to have an ideal smooth surface (Ra = 0 μm). Figure depict the journal bearing layout with a pattern that is only partially rough, while Table reflects the geometry of the bearing and operational conditions studied here.
Table 2. Journal bearing parameter
3.2 Meshing
In this research, the computational domain is constructed using hexahedral elements in ANSYS, which effectively enhances computation efficiency and precision. The refinement indicators, that is, the number of fluid layers in the radial direction, are specified to ensure the accuracy of the results. It is believed that the radial grid is the most essential, as it must be capable of capturing phenomena occurring within the lubricant thickness. Each calculation at the same operating conditions employs a different fluid layer division (and thus different mesh sizes). Figure depicts the results of the grid-independent study in terms of the hydrodynamic pressure under various mesh layers. Based on Figure , the division of lubricating oil layers is determined after sensitivity results indicate that several values of layers division (i.e. 8 and 10) affect the hydrodynamic pressure in the CFD model by less than 4%. In conclusion, the eight layers domain division as depicted in Figure is used for all simulations because it provides a reasonable computational time with a manageable level of independent mesh. This results in the grid distribution of 8 × 360 × 25 in the radial, circumferential, and axial directions. The number of elements in the corresponding computational domain is 72,000, the number of nodes is 84,240, and the maximum skewness is 0.42322.
3.3. Boundary condition and Solution set-up
In this study, the journal moves relative to the stationary bearing surface with the rotational speed ω. Using pressure inlet and pressure outlet boundary conditions, simulations are conducted. The pressure values at the inlet and outlet are assumed to be ambient pressure or zero pressure. The rotating speed of the surface is adjusted at 120 rpm for moving wall boundary conditions. The roughness level Ra for the roughed zone of the bearing varies between 0, 0.4, 1.6, and 12.5 μm. Figure depicts the configuration of boundary conditions for the entire simulation case.
For the velocity—pressure coupling, the SIMPLE scheme is used to obtain an accurate pressure. Regarding spatial discretization, a first-order upwind is utilized for the momentum, turbulent kinetic energy, and turbulent dissipation rate. For volume fraction, the QUICK scheme is selected. The under-relaxation of equations is used by the pressure-based solver to regulate how often computed variables are updated. The default under-relaxation factors for the majority of flows are typically not modified (ANSYS, Citation2019). But in this study, the under-relaxation factors for pressure, momentum, turbulent kinetic energy, and turbulent dissipation rate were decreased from their default values to approximately 0.2, 0,2, and 0.5 for faster convergence.
4. Results
4.1 Validation
This section compares the current numerical results to those obtained from experiments conducted by Wada et al. (Citation1974) and the numerical values provided by Gertzos et al. (Citation2008) in order to validate the developed computational method. It ensures that the computational results are correct and with specified accuracy in the numerical framework. Every situation that arises during the simulation process, notably the bearing with ε = 0.71 and ω = 120 rpm, is the same as in the simulation by Gertzos et al. (Citation2008). displays the validation results in terms of pressure. demonstrates that the difference between the current simulation and a previously published one is approximately 4%, whereas the deviation of the result between the current simulation and the experiment is around 6%. In other words, the values derived using the CFD code produced here are quite close to the published ones, both numerically and experimentally, demonstrating the validity of the current numerical model. It is worth noting that Figure uses the dimensionless parameter as specified in (Gertzos et al., Citation2008) in the manner indicated below:
Figure 6. Comparison between the result of the present investigation and the references (Gertzos et al., Citation2008; Wada et al., Citation1974).

where p denotes the pressure formed on the journal bearing and pa indicates the atmospheric pressure.
4.2 Effect of roughness levels
In this section, the effect of surface roughness level on tribological and noise characteristics is presented in Figure . Here, the bearing with an eccentricity ratio of 0.4 and shaft rotational speed of 120 rpm is of particular interest. In terms of load-carrying capacity, it can be seen in Figure that when the surface roughness is reduced to an ideal level (in this case, Ra = 0), the load-carrying capacity is the highest compared to the other patterns with higher surface roughness. It shows that a partially roughed surface decreases load-carrying capacity. When the surface roughness increases, the load-carrying capacity decreases. For example, from an “ideal” smooth bearing surface to a “fine” surface class, the load-carrying capacity decreases by 84%. While there is a 39% reduction from the “medium” to “rough” level. The reduction of the pressure and thus the load-carrying capacity of the bearing due to roughness as observed in the present results matches well with the finding of Javorova et al. (Citation2016a). As discussed by Javorova et al. (Citation2016b), in the case of bearing lubricated by pseudo-plastic lubricant, the roughness causes maximum pressure and load-carrying capability to decrease.
Figure 7. Effect of surface roughness level on (a) load-carrying capacity, (b) friction force, and (c) average acoustic power level.

Concerning the friction force, compared to the ideal smooth journal bearing, according to Figure , adding roughness at lower levels increases friction force, but as the roughness level is raised to a “rough” level, the friction force gradually starts to decrease. Furthermore, the friction of a roughed bearing with the highest roughness level (i.e. Ra = 12.5 μm) is less than 2% when compared to an ideal smooth bearing, so this difference is not particularly significant. In other words, based on Figure , when the surface is roughed with the highest roughness level (that is, “rough” level in this case), the positive effect can be achieved in comparison to the bearing without roughness. This is understandable given that the oil film thickness increases with surface roughness. According to Yin et al. (Citation2018), this causes the asperity contact to increase and the frictional power loss between the lubricant and the surface to decrease.
In terms of acoustic performance, it is worthwhile to investigate the characteristics of the primary indicator of bearing noise, namely the average acoustic power level. The numerical results show that the roughness lowers the average acoustic power level, as shown in Figure . The acoustic power level decreases as the roughness level is raised. Even though the reduction in bearing noise is not significant (up to 5%), surface modification by adding roughness to the bearing appears to be a promising method of reducing noise. This result is consistent with the findings of F. Meng et al. (Citation2019). It was revealed that as texture or roughness increases, acoustic power decreases. When the surface of the bearing is roughed, it is more difficult for the flow of the lubricant to experience turbulence; as a result, the acoustic magnitude that happens in the fluid is reduced. This is the most likely explanation for the phenomenon. In the future, it will be necessary to conduct a more in-depth study to optimize the roughness, either in roughness shape, the size of the roughed zone, or the optimal value of Ra.
In order to investigate in greater depth the reasons why the tribological performance of the bearing is dependent on the level of surface roughness, the pressure profile, and vapor volume fraction are presented in Figure . The results are analyzed in the mid-plane zone of the bearing with an eccentricity ratio of 0.4 with a journal rotational speed of 120 rpm. Based on Figure , it is observable that the large pressure zone is between 90° and 150°, while the minimal pressure distribution is between 150° and 360°. Furthermore, the “ideal” roughness level of the surface (in this case, Ra = 0 μm) is where the highest pressure is found. While the lowest pressure is reached at Ra = 12.5 μm of roughness (rough). The figure also shows that the expected peak pressure value drops as the roughness rises. Figure further indicates that there is a pressure loss on the journal bearing at an angle of 65°–75°. In detail, Figure shows the contour of the hydrodynamic pressure of the roughed journal bearing for different values of surface roughness level Ra. One can remark from Figure that the rougher the bearing surface, the lower the maximum pressure. Another interesting behavior is that, as shown in Figure , there is a formation of vapor at an angle of 65°–75° in the journal bearing. It also implies that the cavitation model adopted in this study, namely the multiphase mixture cavitation approach, is capable of describing the cavitation processes observed in roughed bearings.
4.3 Effect of eccentricity ratio
Within this section, consideration is given to how the eccentricity ratio influences the performance of bearings. The eccentricity ratio of the bearing, as is well known, occurs as a result of the given loading. Here, as a representation of the loading magnitude, the eccentricity ratio is varied between 0.4, 0.6, 0.71, and 0.8. The primary reason for selecting these numbers is to accommodate the magnitude span of the loading during functioning. For all following simulations, the roughed bearing with a “rough” level is chosen.
Figure depicts the histogram of the tribological (i.e. load-carrying capacity and friction force) and acoustic (i.e. average acoustic power level) indices of the bearing as a function of the eccentricity ratio. From Figure , it can be seen that increasing the eccentricity ratio increases the load-carrying capacity. The friction force follows the same pattern, increasing as the eccentricity ratio does (see Figure . As seen from Figure , for the case of roughed bearing with Bingham plastic lubricant, the tribological performance of bearing at different eccentricity ratios predicted by the present study is in good agreement with the corresponding finding in the available literature (Gertzos et al., Citation2008). Concerning bearing noise, Figure demonstrates that the average acoustic power level increases as the eccentricity ratio increases. The most possible explanation is that as the eccentricity increases, the dispersion of surface acoustic power in the convergent region of the bearing becomes more pronounced, as revealed in Figure . Obviously, this will exacerbate the bearing noise.
5. Conclusion
This study offers a thorough investigation into the tribological and acoustic characteristics of engineered roughed journal bearings lubricated with non-Newtonian Bingham plastic. The analysis focused on key performance parameters like hydrodynamic pressure, load-carrying capacity, friction force, vapor volume fraction, and acoustic power level. In this research, journal bearings with a variety of surface roughness levels were explored. The results were obtained using the computational fluid dynamics (CFD) method including multiphase cavitation. Following are some conclusions that can be reached on the basis of the results and discussion that were presented earlier:
In terms of tribological performance, the introduction of roughness at the leading edge of the surface reduces load-carrying capacity. The addition of roughness greatly lowers the load capacity. In accordance with friction force, the difference between the ideal smooth bearing and the roughed bearing is not that large (about 2%), regardless of the roughness level Ra.
Concerning acoustic behavior, as the roughness level increases, the acoustic power level decreases. Even if the reduction in bearing noise is minor (up to 5%), adding roughness to the bearing appears to be a potential approach to noise reduction.
The eccentricity ratio has a significant impact on both the tribological and acoustic performance of the bearing. The findings of this study are beneficial to the application of designed roughness in enhancing the overall performance of a journal bearing, that is, lowering friction and noise level.
Several limitations in the current roughed journal bearing model may affect the obtained numerical values. First, the current model did not account for the elastic deformation of the bearing components (namely, the journal and the bushing). For future work, the fluid-structure interaction (FSI) method can be utilized to achieve a more precise solution to this problem. Second, in practical applications, thermal loading may impact the bearing’s performance. Unfortunately, this research assumed isothermal conditions. In the future, the energy equation can be incorporated into the calculation to obtain a more comprehensive description of the bearing. In addition, to complement the reference of engineered roughed journal bearing, a more in-depth study will be required to optimize the roughness parameters, either in terms of roughness shape, the size of the roughed zone, or the optimal level of roughness.
Nomenclature
ao | = | Speed of sound [m/s] |
= | Radial clearance [mm] | |
e | = | Eccentricity distance [mm] |
Db | = | Bushing diameter [mm] |
Dj | = | Journal diameter [mm] |
Fcond | = | Condensation coefficient [-] |
Fv | = | Evaporation coefficient [-] |
hmin | = | Minimum fluid film thickness [mm] |
hmax | = | Maximum fluid film thickness [mm] |
k | = | Turbulent kinetic energy [m2/s2] |
ki | = | Consistency index [kg sn-2/m] |
Ks | = | Roughness height [mm] |
= | Bushing length [mm] | |
LRz | = | Roughed surface length [mm] |
Oj | = | Journal center point [-] |
Ob | = | Bearing center point [-] |
n | = | Dimensionless power-law index [-] |
p | = | Hydrodynamic pressure [Pa] |
pv | = | Vapor saturation pressure [Pa] |
Ra | = | Arithmetic mean of roughness (i.e. Roughness level) [ |
RB | = | Bubble radius [mm] |
αv | = | Vapor volume fraction [-] |
αnuc | = | Nucleation volume fraction [-] |
εd | = | Turbulent dissipation rate [m2/s3] |
= | Eccentricity ratio [-] | |
ρ | = | Density of lubricant [kg/m3] |
ρv | = | Vapor density [kg/m3] |
= | Dynamic viscosity of lubricant [Pa.s] | |
= | Yield viscosity [Pa.s] | |
= | Attitude angle [°] | |
= | Circumferential angle [°] | |
= | Shear rate [s−1] | |
= | Yield stress [Pa] | |
= | Shaft rotation speed [rpm] |
Acknowledgments
The authors fully acknowledged Engineering Faculty, Diponegoro University, Directorate of Research and Community Service of Ministry of Research, Technology (DRPM-KEMENRISTEK), and Institute for Research and Community Services (LPPM), Diponegoro University for the approved fund which makes this important research viable and effective.
Disclosure statement
No potential conflict of interest was reported by the authors.
Additional information
Funding
Notes on contributors

Mohammad Tauviqirrahman
Mohammad Tauviqirrahman is Senior Lecturer and Head of Laboratory for Engineering Design and Tribology at the Engineering Faculty, Diponegoro University, Semarang, Central Java, Indonesia. He completed his B.Eng (Diponegoro University, 2003), M.Eng (Institut Teknologi Bandung, 2006) and Doctoral degree (Twente University, the Netherlands, 2013). His research interests include tribology (in lubricant and surface modification), and computational fluid dynamics (CFD). He has published over 86 refereed papers and 676 conference papers. His publications have been cited over 707 times for h-index of 13. As a note, the paper published here is a part of the project “Green Technology for Bearing”, in the roadmap of the research in the Laboratory for Engineering Design and Tribology.
P. Paryanto
P. Paryanto is Head of Production Systems and Industrial Automation Laboratory at the Engineering Faculty, Diponegoro University. He received a doctoral degree from Institute for Factory Automation and Production Systems, University of Erlangen-Nuremberg, Germany.
References
- Abd Al-Samieh, M. F. (2019). Surface roughness effects for newtonian and non-newtonian lubricants. Tribology in Industry, 41(1), 56–16. https://doi.org/10.24874/ti.2019.41.01.07
- Adams, T., Grant, C., & Watson, H. (2012). A simple algorithm to relate measured surface roughness to equivalent sand-grain roughness. International Journal of Mechanical Engineering and Mechatronics (IJMEM), 1, 66–71. https://doi.org/10.11159/IJMEM.2012.008
- ANSYS Inc. (2019). Ansys fluent theory guide 19.0. Canonsburg.
- Bhaskar, S. U., Hussain, M. M., & Ali, M. Y. (2013). Stability analysis on plain journal bearing with effect of surface roughness. International Journal of Scientific & Engineering Research, 4, 1–8.
- Chen, H., & Zhang, Y. (2019). Hydrodynamic journal bearing with slippage sleeve surface. Australian Journal of Mechanical Engineering, 19(2), 1–10. https://doi.org/10.1080/14484846.2019.1587812
- Cui, S., Gu, L., Fillon, M., Wang, L., & Zhang, C. (2018). The effects of surface roughness on the transient characteristics of hydrodynamic cylindrical bearings during startup. Tribology International, 128, 421–428. https://doi.org/10.1016/j.triboint.2018.06.010
- Dang, R. K., Goyal, D., Chauhan, A., & Dhami, S. S. (2020). Effect of non-newtonian lubricants on static and dynamic characteristics of journal bearings. Materials Today: Proceedings, 28, 1345–1349. https://doi.org/10.1016/j.matpr.2020.04.727
- Frene, J., Arghira, M., & Constantinescub, V. (2006). Combined thin-film and Navier–Stokes analysis in high reynolds number lubrication. Tribology International, 39(8), 734–747. https://doi.org/10.1016/j.triboint.2005.07.004
- Gertzos, K. P., Nikolakopoulos, P. G., & Papadopoulos, C. A. (2008). CFD analysis of journal bearing hydrodynamic lubrication by Bingham lubricant. Tribology International, 41(12), 1190–1204. https://doi.org/10.1016/j.triboint.2008.03.002
- Hsu, T. C., Chen, J. H., Chiang, H. L., & Chou, T. L. (2013). Lubrication performance of short journal bearings considering the effects of surface roughness and magnetic field. Tribology International, 61, 169–175. https://doi.org/10.1016/j.triboint.2012.12.016
- Japanese Industrial Standard/Japanese Standards Association JIS B 0601. (2013). Geometrical product specifications (gps)—surface texture: Profile method—terms, definitions and surface texture parameters (foreign standard). Japanese Industrial Standard/Japanese Standards Association. January 2013.
- , J., Mazdrakova, A., Andonov, I., & Radulescu, A. (2016a). Analysis of HD journal bearings considering elastic deformation and non-newtonian Rabinowitsch fluid model. Tribology in Industry, 38, 186–196.
- , J., Stanulov, K., Alexandrov, A., & Iliuta, V. (2016b). Journal bearings lubrication of non-newtonian lubricants with surface roughness effects. Journal of the Balkan Tribological Association, 22, 433–443.
- Kalavathi, G. K., Dinesh, P. A., & Gururajan, K. (2016). Influence of roughness on porous finite journal bearing with heterogeneous slip/no-slip surface. Tribology International, 102, 174–181. https://doi.org/10.1016/j.triboint.2016.05.032
- Kouidera, M., Djallela, Z., Abdelkaderb, Y., & Sahraouia, K. (2021). Mathematical modelling of journal bearing lubricated with non-newtonian fluid. Tribology in Industry, 43, 615–623. https://doi.org/10.24874/ti.1117.05.21.09
- Lampaert, S. G. E., & van Ostayen, R. A. J. (2019). Load and stiffness of a hydrostatic bearing lubricated with a Bingham plastic fluid. Journal of Intelligent Material Systems and Structures, 30(20), 1–10. https://doi.org/10.1177/1045389X19873426
- Lampaert, S. G. E., & van Ostayen, R. A. J. (2020). Lubrication theory for Bingham plastics. Tribology International, 147, 1–7. https://doi.org/10.1016/j.triboint.2020.106160
- Lin, J. R., Chu, L. M., Hung, T. C., & Wang, P. Y. (2016). Derivation of two-dimensional non-newtonian reynolds equation and application to power-law film slider bearings: Rabinowitsch fluid model. Applied Mathematical Modelling, 40(19–20), 8832–8841. https://doi.org/10.1016/j.apm.2016.04.030
- Marey, N., Hegazy, E., El-Gamal, H., Ali, A., & Bassam, A. (2021). Journal bearing performance – state of the art. Sylwan, 165, 390–416.
- Meng, F., Shu, R., & Chen, L. (2020). Influences of operation parameters on noise of journal bearing with compound texture considering lubricant thermal effect. Proceedings of the Institution of Mechanical Engineers, Part J: Journal of Engineering Tribology, 234(7), 991–1006. https://doi.org/10.1177/1350650119868910
- Meng, F., Yu, H., Gui, C., & Chen, L. (2019). Experimental study of compound texture effect on acoustic performance for lubricated textured surfaces. Tribology International, 133, 47–54. https://doi.org/10.1016/j.triboint.2018.12.036
- Meng, F. M., & Zhang, W. (2018). Effects of compound groove texture on noise of journal bearing. Journal of Tribology, 140(3), 031703. https://doi.org/10.1115/1.4038353
- Proudman, I. (1952). The generation of noise by isotropic turbulence. Physical, and Engineering Sciences, 214, 119–132. https://doi.org/10.1098/rspa.1952.0154
- Stout, K. J., & Rowe, W. B. (1974). Externally pressurized bearings — design for manufacture part 1 — journal bearing selection. Tribology International, 7(3), 98–106. https://doi.org/10.1016/0041-2678(74)90009-8
- Tauviqirrahman, M., Afif, M. F., Paryanto, P., Jamari, J., & Caesarendra, W. (2021). Investigation of the tribological performance of heterogeneous slip/no-slip journal bearing considering thermo-hydrodynamic effects. Fluids, 6(2), 48. https://doi.org/10.3390/fluids6020048
- Tauviqirrahman, M., Jamari, J., Susilowati, S., Pujiastuti, C., Setiyana, B., Pasaribu, A. H., & Ammarullah, M. I. (2022). Performance comparison of newtonian and non-newtonian fluid on a heterogeneous slip/no-slip journal bearing system based on CFD-FSI method. Fluids, 7(7), 225. https://doi.org/10.3390/fluids7070225
- Tauviqirrahman, M., Jamari, J., Wicaksono, A. A., Muchammad, M., Susilowati, S., Ngatilah, Y., & Pujiastuti, C. (2021). CFD analysis of journal bearing with a heterogeneous rough/smooth surface. Lubricants, 9(9), 88. https://doi.org/10.3390/lubricants9090088
- Vaz, N., Binu, K. G., Serrao, P., Hemanth, M. P., Jacob, J., Roy, N., & Dias, E. (2017). Experimental investigation of frictional force in a hydrodynamic journal bearing lubricated with magnetorheological fluid. Journal of Mechanical Engineering and Automation, 7, 131–134.
- Wada, S., Hayashi, H., & Haga, K. (1974). Behavior of a Bingham solid in hydrodynamic lubrication: Part 3, application to journal bearing. Bulletin of JSME, 17(111), 1182–1191. https://doi.org/10.1299/JSME1958.16.422
- Walicka, A., Walicki, E., Jurczak, P., & Falicki, J. (2016). Curvilinear squeeze film bearing with rough surfaces lubricated by a Rabinowitsch–Rotem–Shinnar fluid. Applied Mathematical Modelling, 40(17–18), 7916–7927. https://doi.org/10.1016/j.apm.2016.03.048
- Yin, B. B., Zhou, H., Xu, B., & Jia, H. (2018). The influence of roughness distribution characteristic on the lubrication performance of textured cylinder liners. Industrial Lubrication and Tribology, 71(3), 486–493. https://doi.org/10.1108/ILT-07-2018-0258
- Zwart, P., Gerber, A., & Belamri, T. (2004). A two-phase flow model for predicting cavitation dynamics. In: ifth International Conference on Multiphase Flow, Yokohama, Japan, May 30–June3