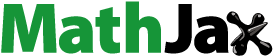
Abstract
Since its inception in the early 1970s by Daniel Gurney, the Gurney flaps have proven to be an effective means of enhancing the aerodynamic performance of an airfoil. Current applications of Gurney flaps include wind turbine blades, race car spoilers, helicopter rotors, and certain high-lift devices. This study compares the influence of Gurney flaps on flow characteristics and the effects of design parameters of the flap, namely the length and position of the flap on a NACA 23,112 airfoil. A review of the studies conducted so far has shown that studies have been carried out on a select few airfoils only and mainly studied the effects of variation of only one of the characteristics, like length, position, or angle. This paper has been crried out to find the optimum balance between these design parameters. Additionally, this paper aims to investigate the accuracy and feasibility of numerical investigation methods to estimate the aerodynamic characteristics of airfoils and wings compared to conventional techniques like wind tunnel testing. This cost-effective computation method is less susceptible to external factors like weather changes. The CFD process also allows testing at conditions that might otherwise be impractical to test in wind tunnels. The workflow comprised a review of literature, validation of solver settings, preparation and testing of the model, extraction of results and analysis of results. Autodesk Fusion360 and Ansys Workbench 22R1 were used to make and test the models. The airfoil designs were tested at Re = 2 × 106, at angles of attack varying from 0° to 15°. The GF height ranged from 1% to 3% chord and placed at 95% to 100% chord. A reliable methodology has been arrived at and validated by implementing the best practices prescribed for CFD. The k- SST turbulence model, along with second-order spatial discretization, was used. Coefficients of lift and drag were considered the study parameters. Gurney flaps were found to increase the performance of the airfoil, and better results were obtained at higher angles of attack. The modified designs noted the separation point further downstream than the baseline model. Overall, the presence of Gurney flaps proved beneficial over the baseline model.
1. Introduction
Lift enhancement devices are used in most aircraft to enhance their aerodynamic efficiency, especially during the takeoff and landing phases. Over the years, designers and engineers have come up with various ingenious ideas to improve the performance of aircraft wings and turbine blades, notably flaps, slats, leading-edge root extensions, co-flow jets, etc. High-lift systems of today can be intricate and are often operated using hydraulics or servos. The most common high-lift equipment in commercial aircraft is flaps and slats, detachable wing components that may be extended to produce extra lift. When deployed, the wing section is altered to improve the camber and surface area. The layout and features of these control interfaces strike a compromise between opposing requirements.
Modern commercial aeroplane wings are designed for effectiveness and optimal speed during cruise flights since the aircraft spends most of its flight time cruising. High-lift devices satisfy this design trade-off by boosting lift during takeoff and landing, decreasing the distance and speed required to land the plane safely, and enabling a more efficient wing in flight.
Devices like the Gurney flaps—simple rectangular plates are attached close to the wing’s trailing edge. They are usually placed on the pressure side of the airfoil, perpendicular to the chord line. The idea of Gurney flaps was first set in motion by Daniel Gurney, an American race car driver and constructor, in 1971 to increase downforce on the inverted wings of a race car. Gurney flaps function by increasing the pressure on the pressure side and decreasing the pressure on the suction side. They also aid in delaying the boundary layer separation up to the trailing edge on the suction side. The Gurney flap increases the airfoil camber by increasing the maximum lift coefficient, lowering zero lift AOA, and raising the nose-down pitching moment. The drag coefficient increases slightly at lower AOA; however, it has been found to reduce drag for thick airfoils. If the flap is appropriately built based on the thickness of the boundary layer, a net improvement in the overall lift-to-drag ratio can be attained.
Blade pitch and twist control, servo flaps, trailing-edge flaps or ailerons, and translational micro tabs can manage wing or rotor blade stresses. Micro tabs or gurney flaps offer the advantages of low actuation power requirements, faster actuation, and less complex operation and manufacturing (Nakafuji et al., Citation2001).
2. Literature review
Since its inception in 1971, the idea of Gurney flaps has been tested and implemented across various applications. Innumerable wind tunnel tests and numerical studies have been carried out with different airfoil configurations and varying design parameters of the flaps themselves. Variations in combinations of other high lift methods like dimples, plasma actuators, multielement airfoils etc., have also been carried out. This section aims to study and list the findings of such studies to pave the way for the paper.
Numerous wind tunnel and numerical analyses were conducted on different airfoil profiles (Liebeck, Citation1978). performed tuft flow visualization of a Newman airfoil, and the results indicated a downwards-turned flow due to the attached flaps. He also found the lift to increase with a minimal drag penalty. Wind tunnel experiments by (Maughmer & Bramesfeld, Citation2008) showed comparable results, where the lift was increased due to increased pressure on the pressure side and shifting of the point of separation of flow aft wards on the suction side. Additionally, it was found that the minimum drag in the case of extended laminar-flow airfoil varies linearly with flap height but is not influenced much by the location of the flaps (Figures ). The aft loading also increases the nose-down pitching moment, resulting from using Gurney Flaps. Aerodynamic characteristics with varying Gurney flap height (Maughmer & Bramesfeld, Citation2008) 25.
Figure 1. Aerodynamic characteristics with a 0.01c-high flap located at the trailing edge on the upper and lower surfaces (Maughmer & Bramesfeld, Citation2008).

(Giguère et al., Citation1995) performed wind tunnel studies for flaps ranging from 0.005c to 0.05c long. Although the lift increment in the 0.05c variant was the highest, the drag penalty was high too. The best aerodynamic was achieved with configurations in the 0.005c to 0.02c range (Neuhart & Pendergraft, Citation1988). obtained similar results with dye flow visualization in a water tunnel. Airfoil pressure distribution revealed that gurney flaps raised the pressure on the pressure side while decreasing the pressure on the suction side compared to a plain version. They deduced that the optimum length of the flap must be close to the boundary layer thickness near the wing’s trailing edge (J. A. C. Kentfield & Clavelle, Citation1993). conducted a detailed study on the physics occurring in the case of Gurney flaps. The following conclusions were drawn: the lift increase due to variation in pressure on either side of the airfoil is valid only for low values of lift coefficient. The increase in lift coefficient increases since adding a gurney flap changes the airfoil’s shape, leading to increased circulation, thus performing better than the baseline airfoil. Another view of interpreting the flow would be that the gurney flaps increase the coefficient of pressure on the pressure side and the circulation of the baseline wing.
(Vuillet et al., Citation1981) conducted tests on a NACA 5414 airfoil with flaps of 1.25% and 5% of chord. The 5% chord flap case increased the lift coefficient by 40% and the lift curve slope by 6%. The zero-lift angle of attack was shifted to −6°. However, the drag coefficient increased considerably for larger sizes of flaps at lower and moderate values of lift coefficients. The 1.25% variant had a much lesser improvement in lift coefficient, but there was no significant drag penalty. The results had a general agreement with the hypothesis of Liebeck (Bloy et al., Citation1997). tested a set of configurations: a 45° wedge and flap, a 90° wedge and flap and a square block as gurney flaps. The 45° flap configuration gave the best results out of the lot. All arrangements provided a significant increase in lift coefficient with a minimal increase in drag. Another variation in design, a T-shaped flap, extended trailing edge and a gurney flap, was tested using CFD. The plain Gurney flap and extended TE flap gave better results when compared to the T-shaped and simple wing variants. Post stall study (Myose et al., Citation1996c) of a NACA 0011 airfoil fitted with a gurney flap showed that despite the stagnation point moving towards the leading edge during a stall, the high pressure remains on the lower side due to the presence of gurney flaps and thus can provide a lift coefficient greater than one even in post-stall regions.
(Jang et al., Citation1998) studied a NACA 4412 airfoil profile fitted with a Gurney flap. The flow field around the airfoil was computed using an incompressible Navier-Stokes algorithm with Gurney flap heights varying from 0.5 to 3.0 % chord. The simulations projected a considerable lift enhancement by introducing a Gurney flap, which improved with flap size but not linearly. Compared to the baseline, the calculated pressure distributions revealed higher loading over the airfoil, notably at the suction peak and towards the trailing edge. At low-to-moderate lift coefficients, the calculations also indicated a drag penalty (Baker et al., Citation2007). performed wind tunnel and computational studies on gurney flaps positioned on the suction and pressure surfaces and found the optimal aerodynamic position for tabs on the pressure side to be at the trailing edge, with a height approximately equal to 2% of the chord (J. A. C. Kentfield, Citation1994). studied the effects of gurney flaps on wind turbines and found a considerable lift coefficient increase for a minimal increase in the drag coefficient (Ion et al., Citation2012). performed a CFD study of T-shaped gurney flaps symmetric along the chord line for application in vertical axis wind turbines. They found that such an arrangement was profitable only when the tip speed ratio of the turbine was high. Studies of three turbine arrays of VAWTs by (Ni et al., Citation2021) showed the flow velocity increase through the gap when the turbines were fitted with Gurney flaps, thus aiding the power output of the downstream rotors, especially for high tip speed ratio cases. For a TSR = 2.27, they achieved an increment of 23.1% in power. Miniature trailing-edge effectors (MiTE) aid in the control of loading on rotorcraft blades. A study by (Kinzel et al., Citation2007) MiTEs showed their potential to improve the performance of rotor blades in high-thrust conditions. A 20% increase in maximum flight speed and a 6% increase in rotor thrust was achieved using MiTEs. These devices are best used to boost capacity and efficiency at high altitudes, with massive payloads, at high speeds or under either of these conditions. MiTEs might also improve cruise performance, as the rotor size could be lowered to boost cruise by enhancing high-lift performance. Unsteady aerodynamics depend heavily on MiTE aerodynamics and surface vortices (Palacios et al., Citation2014). The power consumed by the MiTE was determined and compared to an active plain flap measurement. They appear best suited when devices can provide improved reliability or when deployment techniques favour MiTEs over basic flaps. PIV studies by (Troolin et al., Citation2006) showed two distinct vortex shedding modes, the dominant resembling a Karman vortex shedding and a second caused by intermittent shedding of fluid upstream of the flap. This upstream shedding and its effect on the wake considerably increased lift (Suresh & Sitaram, Citation2011). implemented gurney flaps to study their influence on axial and centrifugal fans. The static efficiency of axial fans was higher than the baseline model, while for centrifugal fans, the fan energy coefficient was notable only at low Reynolds numbers. The static pressure increased in the case of centrifugal fans (Jeffrey et al., Citation2000). performed Laser Doppler Anemometry of an airfoil fitted with gurney flaps. The streamlines showed the formation of a twin vortex structure, and LDA spectral analysis confirmed the presence of alternative shed vortices forming a von Karman vortex. As a result of this shedding, suction at the trailing edge of the airfoil increases. This, in turn, leads to a difference in pressure, ultimately leading to a rise in circulation. As proposed by Liebeck, counter-rotating vortices can be found in the time-averaged flow downstream of the flaps. On the other hand, the instantaneous flow field comprises alternatingly shed vortices. Investigations on the influence of mounting angles and locations of gurney flaps on a NACA0012 airfoil by (Y. Li et al., Citation2003) found flaps perpendicular to the chord line and positioned nearest to the trailing edge gave the best results.
(Traub et al., Citation2006) studied the effects of inclination, porosity, and height variation of gurney flaps on rectangular wings. Flaps inclined at 45° to the surface were found to perform better than perpendicular flaps with the same projected size. However, an increase in porosity decreased the performance of the flaps (Gai & Palfrey, Citation2003). and (van Dam et al., Citation1999) conducted comparative studies on solid and serrated flaps, and the former was more efficient. In the case of delta wings (Y. C. Li et al., Citation2002), porosity in the flaps was more favourable, with a 71% increase in lift coefficient for a serrated flap configuration. The solid flap configuration gave only a maximum increment of 51.4%. Another interesting aspect of the study also revealed that the advantages of gurney flaps were reduced when the wing was sideslipped (Shukla & Kaviti, Citation2017). studied effects of dimples near the trailing edge of the airfoil in addition to gurney flaps. The dimples were situated adjacent to the flaps upstream. Results showed that the configuration with only Gurney flaps was the most effective in increasing lift compared to designs with only a dimple and both dimple and flap.
Experiments were carried out on a multielement airfoil having gurney flaps on the trailing element (Katz & Largman, Citation1989; Papadakis et al., Citation1996, Citation1997), the primary element alone (Papadakis et al., Citation1996, Citation1997; Ross et al., Citation1995), or on both (Papadakis et al., Citation1996, Citation1997; Storms & Ross, Citation1995). The performance was enhanced in the case of flaps on the trailing element but not in the case of the flaps on the main element (Myose et al., Citation1996, Citation1998) experimented on three-dimensional cambered wings with gurney flaps with single and multiple elements. The angle of attack for zero lift increases negatively, and the lift curve shifts upwards in the presence of the flaps. Although the stall angle reduces with increased flap height, the maximum lift coefficient increases. A 25%, 36% and 47% increase were reported with flap heights of 0.01c, 0.02c and 0.04c, respectively. For the cambered case, a 22% increase in lift coefficient was obtained for a flap of 0.01c height. Their study of three-dimensional wings found that the shape of the lift-curve slope is altered totally due to gurney flaps, as opposed to a mere shift in the curve for two-dimensional cases. Also, positioning the flaps in the inboard position is more favourable than the outboard position. A study by (Storms & Jang, Citation1994) on a NACA4412 airfoil with vortex generators and Gurney flaps showed that the two devices could be used to yield better results. The combination’s lift-to-drag ratio was higher at higher AOA but lower at moderate and low AOA. As a result, vortex generators can be hidden within high-lift devices to decrease parasitic drag during cruising mode (J. Kentfield, Citation1993). investigated the use of Gurney flaps on helicopter rotors and discovered a 20% improvement in the lift with no extra power or propeller diameter increase. The overall efficiency increases by 10% with the addition of flaps. With a focus on improving the lifting capability of an airfoil with a minimal penalty, Gurney flaps could be an ideal choice. The geometry and deployment methods are simple to achieve. Gurney flaps enhance the lift coefficient with minimum drag penalty by enhancing airfoil circulation, delaying flow separation, and raising the pressure coefficient ahead on the pressure side. The lift coefficient remains near or over-unity due to the high pressure on the pressure side post-stall, which might be helpful for aviation applications. Gurney flaps reduce the power consumed by a considerable amount for application in compressor blades. Applications in helicopter blades can also enhance the flight envelope and increase payload carrying capacity. Wind turbine applications of gurney flaps can be beneficial to decrease starting speed when used near the root, controlling loads by deflectable flaps, combined with vortex generators to adapt to high-power machinery (Salcedo et al., Citation2006) etc.
Recent applications of Gurney flaps are primarily found in VAWTs (Syawitri et al., Citation2022). used a hybrid transition SST model along with the Taguchi method to study the performance of VAWTs fitted with Gurney flaps at different TSRs. While the flaps increased the power coefficient at lower TSRs, a decreasing trend was found with increasing TSR. Another study by (Zhu et al., Citation2019) consisted of varying positions of Gurney flaps (inward, outward, and on both sides) and GFs with dimples. While the outboard dimple GF saw a 17.92% increase in power coefficient at a TSR of 3.1, the other models appeared more advantageous than the baseline model. On the other hand, intense interaction among the blades constrains the number of blades(n), with maximum performance obtained at n = 5. The effectiveness of Gurney flaps on Darrieus wind turbines was studied (Bianchini et al., Citation2019; Yan, Avital, Williams, & Korakianitis, Citation2019). The results were consistent with other studies of similar nature. Gurney flaps on the Wells turbine were tested, and evidence of an increased torque coefficient of 81% was obtained (Kotb et al., Citation2022). The k-ω SST turbulence model was utilized along with second-order upwind discretization schemes.
However, there have been cases where the addition of gurney flaps has reduced the maximum lift coefficient (Baker et al., Citation2007; Cole et al., Citation2013). This was mainly found in the aft-loaded airfoil. The reason is that Gurney flaps negate the lift produced by the airfoil. A NACA 23,112 airfoil is studied in this paper, and results are reported considering such possibilities. Also, most of the previous studies (Yan, Avital, Williams, & Korakianitis, Citation2019), (Yan, Avital, Williams, & Cui, Citation2019), have been carried out to investigate the effect of one of the parameters of the flap (either length, position etc.). The current study aims to capture the impact of such parameters collectively. To conclude, this paper uses a modern study method—CFD – to test the suitability of Gurney flaps and the consequences of varying its parameters on a NACA 23,112 airfoil.
3. Methodology
This section investigates the aerodynamic behaviour of the airfoil with Gurney flaps installed. NACA 23,112 profile was chosen for the study. Numerical validation and mesh independence studies were carried out to ensure the accuracy of the results obtained using the CFD solver. The computation was carried out using commercial CFD software—Ansys Fluent, with the parameters as mentioned in Table . Computational methods are used to calculate the values used in the analysis. The simulation’s outcomes can also be mathematically validated or applied. The lift and drag coefficients (EquationEquation 1(1)
(1) and Equation2
(2)
(2) ) are used to evaluate the findings.
Table 2. Geometric and operational parameters
3.1. Geometry and computational domain
The airfoil chord length is set to be 1 m. The selected airfoil profile NACA 23,112 is modelled using Autodesk Fusion360. The modifications carried out (Figure ) are i) variation in positioning of the flap “p” from LE of the airfoil (0.95 c to 1.0 c) ii) variation of the height “h” of the flap (0.01c to 0.03c). Previous studies of other airfoils (Y. Li et al., Citation2002; Myose et al., Citation1996a; Windi et al., Citation2014) show that the flap’s optimum length is close to 2% of the chord length. The thickness of the flaps is maintained at 2 mm. While conducting the study, the angle of attack, i.e., the angle between the free-stream velocity and the chord line, varied from 0 to 15 degrees.
The computational domain is set per standard best practices, with sufficient area in the vicinity and far-field of the flow, ensuring that boundary effects do not influence the results. The face-splitting function divides the domains for extended local mesh size control. The dimensions of the domain are shown in Figure . With the airfoil in the centre; a domain is built around the airfoil on which boundary conditions are applied, resulting in a full atmospheric setup. A surface-based design without any thickness is carried out to show the improved benefits and detailed results.
3.2. Meshing and grid sensitivity analysis
Non-structured grid is used to build the mesh for the setup. Body and edge sizing is set to the required sizes to ensure a fine mesh surrounding the airfoil. The boundary layer has been incorporated using a series of 20 inflation layers, with the first layer thickness equal to 1.2 × 10−5 m, to obtain a Y+ < 1, where Y+ is the dimensionless wall distance between the first layer of the grid and the wall. The element type is set to quadrilateral dominant. The number of elements is of the order of 2.12 × 106 elements. The maximum skewness is within 0.80, and the average is around 0.17.
Three mesh combinations were tested for sensitivity analysis, M1, M2 and M3 (Table ) at v = 32.99 m/s and k- SST turbulence model. The M2 configuration with 2.12 × 106 elements was selected for the study as further refinement only increased computational load and showed little error-value change.
Table 1. Mesh sensitivity analysis
3.3. Solver setup
The energy model is used as it combines different conditions and parameters for solving the problem. The conservation laws, namely mass, momentum, and energy, are incorporated using the appropriate model settings. The study is conducted at an air density of 1.184 kg/m3 and dynamic viscosity of 1.86 × 10−5 N s/m2.
3.4. Boundary conditions
Velocity inlet and pressure outlet conditions are used in the study (Figure ). Inlet velocity is set to 32.99 m/s resulting in Reynold’s number of 2 × 106. Turbulence intensity was calculated to be 0.45% with a length scale of 0.07. The outlet was set to a pressure outlet with a gauge pressure of 102,036.05 Pa. The domain walls were set to zero shear, while the wing surface was set to no slip.
3.5. Turbulence model
The k- SST turbulence model, developed by (Menter, Citation1994), is used for the study. Numerous studies (Balduzzi et al., Citation2017; Belabes & Paraschivoiu, Citation2021; Ji et al., Citation2022; Kotb et al., Citation2022) have shown that this model can accurately mimic the flow in the case of wind turbines utilizing its hybrid properties. The shear-stress transport (SST) k-
model successfully integrates the near-wall region’s accurate and effective formulation of the k-
model with the distant field’s free-stream independence.
The SST model is more accurate for a broader range of flows like flow around an airfoil, adverse pressure gradient flow, transonic shocks etc. This model has been reported to provide expected results in 2D and 3D simulations of turbulent flow over an airfoil (Bartl et al., Citation2019; Cakmakcioglu et al., Citation2014; Yang et al., Citation2014). The transport equations (k-w SST turbulence model, Citation2022) for k and are given by EquationEquation 3
(3)
(3) and Equation4
(4)
(4) .
A comparison study by (Huang et al., Citation2021) showed that the k- SST model performed considerably better than the k- ℇ model, and the results agreed with experimental results. Similar results were obtained in several other studies containing flow over an airfoil (Ahmad et al., Citation2005; Meana-Fernández et al., Citation2019; Ouchene et al., Citation2018; Zhang et al., Citation2020). Hence, this study uses the k-
SST turbulence model. Best practices for numerical simulations have been used as recommended in the previous literature.
3.6. Validation of solver model
The results obtained from the model are compared to those from experimental studies. First, a plain NACA 23,112 airfoil was tested, and the results were in accordance with the literature (Jacobs & Pinkerton, Citation1936). (Figures ). Further, an S903 (12%) airfoil was tested with a 2%c Gurney flap at 100%c at Re = 106, and the results had minimal deviations (Figure ) from experimental studies by (Maughmer & Bramesfeld, Citation2008).
Figure 4. Validation plot of Cl with experimental data (Jacobs & Pinkerton, Citation1936).

Figure 5. Validation plot of Cd with experimental data (Jacobs & Pinkerton, Citation1936).

Figure 6. Comparison of Cl values obtained from CFD and experimental data (Maughmer & Bramesfeld, Citation2008).

The airfoil geometry is modelled as a surface using Autodesk Fusion360 and then imported to ANSYS DesignModeler. A domain is built around the airfoil surface, and the profile is Boolean subtracted from the domain. Further, ANSYS meshing creates a mesh, with more refined elements near the airfoil being made using split faces. Edge sizing is used on the airfoil to control the size of the elements. An inflation layer is used to separate the domain and the airfoil. All zones are set to fluid, and a mesh is generated. Checks are performed to ensure that results are independent of mesh quality. The mesh is then imported to ANSYS Fluent, where a density-based solver is used. K-w SST turbulence model is implemented, and boundary conditions are set.
Monitors are set to ensure results are within an acceptable range. The second-order upwind scheme is used to discretize flow, turbulent kinetic energy and specific dissipation rate and iterated until convergence of 10−6 is obtained with lift and drag coefficients. A detailed workflow is shown in Figure
4. Result analysis
The various models tested were compared for aerodynamic efficiency with the plain model of the NACA23112 airfoil. The various parameters tested have been graphically represented in this chapter. Contours of pressure and velocity for select configurations have also been provided to visualize the flow. The models tested are X-YY, where X is the flap height as a percentage of the chord, and YY is the position of the flap from LE as a percentage of the chord.
4.1. Effect of flap height and position
Evaluation of the graphs in Figure shows an increase in the lift coefficient of the airfoil with increasing flap height. While the 1%c high flap provided a 60% increase in Cl, the 2%c and 3%c models provided a maximum increment of 98% and 130% in Cl. However, this lift increase came with an increased drag component, with a 52%, 94% and 153% increase in the drag coefficient (Figure ). This increase in drag varies linearly with the rise in flap height. (Figure )
Figure 8. (A) Cl vs AoA 1-YY, (B) Cl vs AoA 2-YY, (C) Cl vs AoA 3-YY, (C) Comparison of Cl among best configurations with 1, 2 and 3% c flaps.

Figure 9. (A) Cd vs AoA 1-YY, (B) Cd vs AoA 2-YY, (C) Cd vs AoA 3-YY, (C) Comparison of Cd among best configurations with 1, 2 and 3% c flaps 33.

While comparing the effects of the position of the flaps, those situated on the trailing edge were found to be most beneficial. A maximum increase of 125%, 129% and 135% was noted for Cl of the X−95, X−97, and X−100 configurations, respectively, while a rise of 153%, 147% and 140% were found in their drag coefficients. Also notable is the premature onset of stall using Gurney flaps. However, the Cl corresponding to these AoAs, is comparatively higher than the pain wing, thus allowing for easier stall recovery.
To evaluate the best variant of those tested, the Cl/Cd values of the designs were compared (Figure ). The 1–100 variant was found to have the best increase in aerodynamic efficiency over the range of AoAs tested, with an increase of 37% in Cl/Cd at 14°. The maximum increment in Cl/Cd for each variant has been tabulated below (Table ).
Figure 11. (A) Cl/Cd vs AoA 1-YY, (B) Cl/Cd vs AoA 2-YY, (C) Cl/Cd vs AoA 3-YY, (C) Comparison of Cl/Cd among best configurations with 1, 2 and 3% c flaps.

Table 3. Maximum values of Cl/Cd for each configuration
The pressure on the lower side of the airfoil is higher in the case of wings with flaps, which leads to an increased lift due to the flaps. A slight increase in the maximum pressure can also be observed from the contours (Figure )
Figure 12. Pressure contours for (A) Plain wing at 10° AoA (B) TE of Plain wing at 10° AoA (C) 1–95 at 10° AoA (D) TE of 1–95 at 10° AoA (E) 2–95 at 10° AoA (F) TE of 2–95 at 10° AoAA.

As seen from the velocity contours (Figure ), the point of separation of flow moves further downstream on the suction side of the airfoil. This helps increase the lift obtained by the airfoil due to increased circulation. This behaviour aligns with the expected results of various numerical and experimental studies.
5. Discussion and conclusions
The current paper will study the effect of gurney flaps on the aerodynamic performance of a NACA23112 reflex airfoil with a 1 m chord. The main objective of this paper is to study the effect of three different flap lengths and positions on the aerodynamic performance of the airfoil. CFD method using Ansys Fluent has been adopted for the project. The models and settings used have been validated with results from experimental studies conducted by other researchers, and mesh independence studies have been carried out. The lift and drag coefficients are reported, which are used to arrive at the aerodynamic efficiency. Further, the models are referred to as X-YY, where X is the length of the flap percentage of the chord, and YY is the position of the flap from the leading edge of the airfoil as a percentage of the chord. The following conclusions were drawn.
Gurney flaps aid in increasing the lift and drag coefficients of the airfoil. This increase is related to both the height and position of the flap.
With an increase in the flap height from 1% to 3% chord, the lift coefficient increases from 60% to 130%. However, the drag coefficient also increased from 52% to 153%, with the rise in flap height. A balance is thus necessary to obtain optimal performance from the Gurney flaps.
Among the three positions tested, the increase in Cl varied from 125% to 135%, and the increase in Cd ranged from 153% to 140% for the configurations. The drag was less affected by the change in the position of the flap as opposed to the effect of its length.
The designs’ aerodynamic efficiency (Cl/Cd) was compared to arrive at the best configuration. A 37.9% increase in Cl/Cd was obtained for the 1-100 design. This configuration also had the best efficiency throughout the entire range of angles of attack tested.
The premature onset of the stall was noted due to the use of Gurney flaps. While the stall angle of the plain wing was nearly 18°, the wing with GFs had a stall angle close to 14°. However, the lift coefficient during stall was higher than the plain wing, thus resulting in better stall recovery.
The pressure and velocity contours confirmed the increase in pressure on the pressure side and the delay of the point of separation of the flow on the suction side near the trailing edge. This agrees with previous findings on the flaps’ working principle.
To conclude, the lift and drag coefficients observe an expected general trend. The overall efficiency of the airfoil increased, especially at higher angles of attack for most of the configurations, as seen in the plots. The best position to place the flaps was the 1–100 configuration consisting of a 1%c flap on the airfoil’s trailing edge. Previous studies (Y. Li et al., Citation2002; Myose et al., Citation1996b; Neuhart & Pendergraft, Citation1988; Windi et al., Citation2014) have suggested that the optimal value of the height of the flap be equal to the boundary layer thickness near the trailing edge. Investigating the performance of the flaps, we can also deduce the boundary layer thickness to be in the range of 1–2%c near the TE for NACA 23,112.
Higher values of Cl are also attained post-stall using Gurney flaps, which can benefit aircraft during stall recovery. These flaps require minimal deployment mechanisms and thus reduce the manufacturing complexion and weight of the aircraft. The flaps are also advantageous in the case of HAWTs and VAWTs, enabling operation at lower wind speeds. The flaps’ increased performance easily overcomes the minimal drag penalty. In addition, the numerical computation method can be used to perform studies in conditions that are otherwise arduous to perform, like in case of higher velocities, and results are prone to changes due to external factors like weather conditions, wind tunnel equipment etc. With developments in computational methods, time and resources can be utilized more economically.
A future study could be carried out on implementing NACA 23,112 airfoil with GFs in VAWTs and HAWTs. The effect of serrations, vanes, variation of flap angles, etc., can be studied. As the flaps tend to deteriorate performance downstream in the case of VAWTs, mechanisms to retract and deploy the flaps during operation can prove helpful.
Nomenclature
c | = | chord length |
Cl | = | Coefficient of lift |
Cd | = | Coefficient of drag |
D | = | Drag force |
HAWT | = | Horizontal axis wind turbine |
L | = | Lift force |
LDA | = | Laser Doppler anemometry |
LE | = | Leading edge |
MiTE | = | Miniature trailing edge |
TE | = | Trailing edge |
TSR | = | Tip speed ratio |
Vair | = | free-stream velocity |
VAWT | = | Vertical axis wind turbine |
= | generation of turbulence kinetic energy due to the mean velocity gradients | |
= | effective diffusivity of k and | |
ρair | = | Density of air |
Yk and Yω | = | turbulent dissipation of k and ω |
Dω | = | cross-diffusion terms |
Sk and Sω | = | user-defined source terms. |
X-YY | = | length of the flap in mm and position of the flap from LE as a percentage of chord |
Acknowledgments
Firstly, we would like to thank Dr Anil Rana, Director, Manipal Institute of Technology, Manipal, for providing the necessary support and infrastructure to carry out this project. We would also like to extend our heartiest gratitude to Dr Dayananda Pai K, Head of the Department of Automobile and Aeronautical Engineering, Manipal Institute of Technology, Manipal, for providing us with the opportunity and necessary guidance to undertake this study.
Disclosure statement
No potential conflict of interest was reported by the authors.
Additional information
Funding
Notes on contributors
Vandan Chinnappa
Vandan Chinnappa—worked on conceptualization, methodology, investigation, numerical study, and writing—original draft.

Srinivas G
SrinivasG is a faculty in the Department of Aeronautical and Automobile engineering at Manipal Institute of Technology – Manipal Academy of Higher Education (MAHE). He is a doctorate from Manipal Academy of Higher Education –MAHE (Institute of Eminence)- Manipal, India. He is experienced in the field of Aircraft aerodynamics, Aircraft Propulsion, Rocket and Missiles Aerodynamics, Air Transportation system, computational fluid mechanics and has published more than 40+ articles in leading Aerospace and Mechanical Science related journals. He has mentored several UG and Graduate students and is the Faculty Advisor of MIT’s collegiate and one of the world’s leading student sounding rocketry design and Research club “thrustMIT”. He is an institute coordinator and member of prestigious Institution of Power Engineers (IPowerE). His research interest includes Aircraft Propulsion, Rocket Propulsion, Aerodynamics of Rockets and Missiles, Aircraft Design, Computational Fluid Dynamics, Mechanics of Fluids and Aerodynamics, and is currently exploring topics related to Transonic flow and Hypersonic flow regimes applications.
References
- Ahmad, K. A., Mcewan, W., Watterson, J. K., and Cole, J., “RANS turbulence models for pitching airfoil,” 2005. [Online]. Available https://www.witpress.com
- Baker, J. P., Standish, K. J., & van Dam, C. P. (2007). Two-dimensional wind tunnel and computational investigation of a Microtab Modified Airfoil. Journal of Aircraft, 44(2), 563–19. https://doi.org/10.2514/1.24502
- Balduzzi, F., Drofelnik, J., Bianchini, A., Ferrara, G., Ferrari, L., & Campobasso, M. S. (2017, June). Darrieus wind turbine blade unsteady aerodynamics: A three-dimensional Navier-Stokes CFD assessment. Energy, 128, 550–563. https://doi.org/10.1016/J.ENERGY.2017.04.017
- Bartl, J., Sagmo, K. F., Bracchi, T., & Sætran, L. (2019, May). Performance of the NREL S826 airfoil at low to moderate Reynolds numbers—A reference experiment for CFD models. European Journal of Mechanics - B/fluids, 75, 180–192. https://doi.org/10.1016/J.EUROMECHFLU.2018.10.002
- Belabes, B., & Paraschivoiu, M. (2021, October). Numerical study of the effect of turbulence intensity on VAWT performance. Energy, 233, 121139. https://doi.org/10.1016/J.ENERGY.2021.121139
- Bianchini, A., Balduzzi, F., diRosa, D., & Ferrara, G. (2019, March). On the use of Gurney Flaps for the aerodynamic performance augmentation of Darrieus wind turbines. Energy Conversion and Management, 184, 402–415. https://doi.org/10.1016/J.ENCONMAN.2019.01.068
- Bloy, A. W., Tsioumanis, N., & Mellor, N. T. (1997). Enhanced aerofoil performance using small trailing-edge flaps. Journal of Aircraft, 34(4), 569–571. https://doi.org/10.2514/2.2210
- Cakmakcioglu, S. C., Sert, I. O., Tugluk, O., & Sezer-Uzol, N. (2014, June). 2-D and 3-D CFD Investigation of NREL S826 airfoil at low Reynolds numbers. Journal of Physics: Conference Series, 524, 12028. https://doi.org/10.1088/1742-6596/524/1/012028
- Cole, J. A., Vieira, B. A. O., Coder, J. G., Premi, A., & Maughmer, M. D. (2013). Experimental investigation into the effect of gurney flaps on various airfoils. Journal of Aircraft, 50(4), 1287–1294. https://doi.org/10.2514/1.C032203
- Gai, S. L., & Palfrey, R. (2003, March). Influence of trailing-edge flow control on airfoil performance. Journal of Aircraft, 40(2), 332–337. https://doi.org/10.2514/2.3097
- Giguère, P., Lemay, J., and Dumas, G., “Gurney flap effects and scaling for low-speed airfoils,” In 13th Applied Aerodynamics Conference, 1995, pp. 966–976. https://doi.org/10.2514/6.1995-1881.
- Huang, B., Wang, P., Wang, L., Cao, T., Wu, D., & Wu, P. (2021, December). A combined method of CFD simulation and modified Beddoes-Leishman model to predict the dynamic stall characterizations of S809 airfoil. Renewable Energy, 179, 1636–1649. https://doi.org/10.1016/J.RENENE.2021.07.131
- Ion, M., Radu, B., & Horia, D. (2012, December). Theoretical performances of double Gurney flap equipped the VAWTs. INCAS BULLETIN, 4(4), 93–99. https://doi.org/10.13111/2066-8201.2012.4.4.8
- Jacobs, E. N. and Pinkerton, R. M., (1936). “NACA-Report 537.”
- Jang, C. S., Ross, J. C., & Cummings, R. M. (1998). Numerical investigation of an airfoil with a Gurney flap. Aircraft Design, 1(2), 75–88. https://doi.org/10.1016/S1369-8869(98)00010-X.
- Jeffrey, D., Zhang, X., & Hurst, D. W. (2000). Aerodynamics of Gurney flaps on a single-element high-lift wing. Journal of Aircraft, 37(2), 295–301. https://doi.org/10.2514/2.2593
- Ji, B., Zhong, K., Xiong, Q., Qiu, P., Zhang, X., & Wang, L. (2022, June). CFD simulations of aerodynamic characteristics for the three-blade NREL Phase VI wind turbine model. Energy, 249, 123670. https://doi.org/10.1016/J.ENERGY.2022.123670
- Katz, J., & Largman, R. (1989, April). Effect of 90 degree flap on the aerodynamics of a two-element airfoil. Journal of Fluids Engineering, Transactions of the ASME, 111(1), 93–94. https://doi.org/10.1115/1.3243605
- Kentfield, J. (1993). The potential of Gurney flaps for improving the aerodynamic performance of helicopter rotors. Dec. https://doi.org/10.2514/6.1993-4883
- Kentfield, J. A. C. (1994). Theoretically and experimentally obtained performances of Gurney-flap equipped wind turbines. Wind Engineering, 18(2), 63–74.
- Kentfield, J. A. C., & Clavelle, E. J. (1993). The flow physics of Gurney flaps, devices for improving turbine blade performance. Wind Engineering, 17(1), 24–34.
- Kinzel, M. P., Maughmer, M. D., & Lesieutre, G. A. (2007). Miniature trailing-edge effectors for rotorcraft performance enhancement. Journal of the American Helicopter Society, 52(2), 146–158.
- Kotb, A. T. M., Nawar, M. A. A., Attai, Y. A., & Mohamed, M. H. (2022, September). Performance assessment of a modified wells turbine using an integrated casing groove and Gurney flap design for wave energy conversion. Renew Energy, 197, 627–642. https://doi.org/10.1016/J.RENENE.2022.07.140
- “k-w SST turbulence model.” (Retrieved March 6, 2022). https://doi.org/10.2514/3.58406/
- Liebeck, R. H. (1978, September). Design of subsonic airfoils for high lift. Journal of Aircraft, 15(9), 547–561.
- Li, Y. C., Wang, J. J., Tan, G. K., & Zhang, P. F. (2002). Effects of Gurney flaps on the lift enhancement of a cropped nonslender delta wing. Experiments in Fluids, 32(1), 99–105. https://doi.org/10.1007/s003480200010
- Li, Y., Wang, J., & Zhang, P. (2002). Effects of Gurney flaps on a NACA0012 airfoil. Flow Turbulence & Combustion, 68(1), 27. https://doi.org/10.1023/A:1015679408150
- Li, Y., Wang, J., & Zhang, P. (2003). Influences of mounting angles and locations on the effects of Gurney flaps. Journal of Aircraft, 40(3), 494–498. https://doi.org/10.2514/2.3144
- Maughmer, M. D., & Bramesfeld, G. (2008). Experimental investigation of Gurney flaps. Journal of Aircraft, 45(6), 2062–2067. https://doi.org/10.2514/1.37050
- Meana-Fernández, A., Fernández Oro, J. M., Argüelles Díaz, K. M., & Velarde Suárez, S. (2019). Turbulence-model comparison for aerodynamic-performance prediction of a typical vertical-axis wind-turbine airfoil. Energies (Basel), 12(3), 488. https://doi.org/10.3390/en12030488
- Menter, F. R. (1994, August). Two-equation eddy-viscosity turbulence models for engineering applications. AIAA Journal, 32(8), 1598–1605.
- Myose, R., Heron, I., & Papadakis, M. (1996a). Effect of gurney flaps on a NACA 0011 airfoil. https://doi.org/10.2514/6.1996-59
- Myose, R., Heron, I., & Papadakis, M. (1996b). Effect of Gurney flaps on a NACA 0011 airfoil. In 34th Aerospace Sciences Meeting and Exhibit. American Institute of Aeronautics and Astronautics. https://doi.org/10.2514/6.1996-59/
- Myose, R., Heron, I., & Papadakis, M. (1996c). The post-stall effect of Gurney flaps on a NACA-0011 airfoil. SAE Transactions, 105, 173–178. [Online]. Available. http://www.jstor.org/stable/44725501
- Myose, R., Papadakis, M., & Heron, I. (1998). Gurney flap experiments on airfoils, wings, and reflection plane model. Journal of Aircraft, 35(2), 206–211. https://doi.org/10.2514/2.2309
- Myose, R., Papadakis, M., Heron, I., & Angeles, L. (1996). The effect of Gurney flaps on three dimensional wings with and without taper. SAE Technical Paper, 965514. https://doi.org/10.4271/965514
- Nakafuji, D. T. Y., van Dam, C. P., Smith, R. L., & Collins, S. D. (2001, July). Active load control for airfoils using microtabs. Journal of Solar Energy Engineering, 123(4), 282–289. https://doi.org/10.1115/1.1410110
- Neuhart, D. H., & Pendergraft, O. C. (1988). A water tunnel study of Gurney flaps. NASA TM-4071. https://ntrs.nasa.gov/citations/19890004024
- Ni, L., Miao, W., Li, C., & Liu, Q. (2021). Impacts of Gurney flap and solidity on the aerodynamic performance of vertical axis wind turbines in array configurations. Energy, 215, 118915. https://doi.org/10.1016/j.energy.2020.118915
- Ouchene, S., Smaili, A., & Fellouah, H. (2018). Numerical simulation of a pitching NACA 0015 airfoil in deep stall regime: Comparison of turbulence models. 2018 International Conference on Wind Energy and Applications in Algeria (ICWEAA) (pp. 1–7). https://doi.org/10.1109/ICWEAA.2018.8605100
- Palacios, J., Kinzel, M., Overmeyer, A., & Szefi, J. (2014). Active Gurney flaps: Their application in a rotor blade centrifugal field. Journal of Aircraft, 51(2), 473–489. https://doi.org/10.2514/1.C032082
- Papadakis, M., Myose, R., Heron, I., and Johnson, B., “An experimental investigation of Gurney flaps on a GA(W)-2 airfoil with 25 percent slotted flap,” in 14th Applied Aerodynamics Conference, American Institute of Aeronautics and Astronautics, 1996. https://doi.org/10.2514/6.1996-2437.
- Papadakis, M., Myose, R., Matallana, S., Papadakis, M., Myose, R., & Matallana, S. (1997). Experimental investigation of Gurney flaps on a two element general aviation airfoil. In 35th Aerospace Sciences Meeting and Exhibit. American Institute of Aeronautics and Astronautics. https://doi.org/10.2514/6.1997-728
- Ross, J. C., Storms, B. L., & Carrannanto, P. G. (1995, May). Lift-enhancing tabs on multielement airfoils. Journal of Aircraft, 32(3), 649–655. https://doi.org/10.2514/3.58406
- Salcedo, S., Monge, F., Palacios, F., Gandia, F., Rodriguez, A., & Barcala, M. (2006, January). Gurney flaps and trailing edge devices for wind turbines. EWEC. Athens: EWEA, 2(64), 1180–2, 1184. https://www.researchgate.net/publication/291146203_Gurney_flaps_and_trailing_edge_devices_for_wind_turbines
- Shukla, V., & Kaviti, A. K. (2017). Performance evaluation of profile modifications on straight-bladed vertical axis wind turbine by energy and spalart allmaras models. Energy, 126, 766–795. https://doi.org/10.1016/j.energy.2017.03.071
- Storms, B. L., & Jang, C. S. (1994). Lift enhancement of an airfoil using a Gurney flap and vortex generators. Journal of Aircraft, 31(3), 542–547. https://doi.org/10.2514/3.46528
- Storms, B. L., & Ross, J. C. (1995, September). Experimental study of lift-enhancing tabs on a two-element airfoil. Journal of Aircraft, 32(5), 1072–1078.
- Suresh, M., & Sitaram, N. (2011). Gurney flap applications for aerodynamic flow control. Dec, ICME2011. https://me.buet.ac.bd/icme/icme2011/Proceedings/PDF/ICME%2011-FL-040.pdf
- Syawitri, T. P., Yao, Y., Yao, J., & Chandra, B. (2022). Geometry optimization of vertical axis wind turbine with Gurney flap for performance enhancement at low, medium and high ranges of tip speed ratios. Sustainable Energy Technologies and Assessments, 49, 101779. https://doi.org/10.1016/j.seta.2021.101779
- Traub, L. W., Miller, A. C., & Rediniotis, O. (2006). Preliminary parametric study of Gurney flap dependencies. Journal of Aircraft, 43(4), 1242–1244. https://doi.org/10.2514/1.13852
- Troolin, D. R., Longmire, E. K., & Lai, W. T. (2006, August). Time resolved PIV analysis of flow over a NACA 0015 airfoil with Gurney flap. Experiments in Fluids, 41(2), 241–254. https://doi.org/10.1007/s00348-006-0143-8
- van Dam, C. P., Yen, D. T., & Vijgen, P. M. H. W. (1999). Gurney flap experiments on airfoil and wings. Journal of Aircraft, 36(2), 484–486. https://doi.org/10.2514/2.2461
- Vuillet, A., Roesch, P., & Aerospatiale Helicopter Divison. (1981). New designs for improved aerodynamic stability on recent aerospatiale helicopters. Proceedings of the 12th European Rotorcraft Forum, Garmisch-Partenkirchen, Germany.
- Windi, I. S., Faris, M., & Kareem, H. H. (2014). Experimental and theoretical investigation for the improvement of the aerodynamic characteristic of NACA 0012 airfoil. International Journal of Mining, Metallurgy & Mechanical Engineering, 2(1). https://api.semanticscholar.org/CorpusID:212554656?utm_source=wikipedia
- Yan, Y., Avital, E., Williams, J., & Cui, J. (2019, October). Performance improvements for a vertical axis wind turbine by means of Gurney flap. Journal of Fluids Engineering, 142(2), https://doi.org/10.1115/1.4044995
- Yan, Y., Avital, E., Williams, J., & Korakianitis, T. (2019, May). CFD analysis for the performance of Gurney flap on aerofoil and vertical axis turbine. International Journal of Mechanical Engineering and Robotics Research, 8(3), 385–392. https://doi.org/10.18178/ijmerr.8.3.385-392
- Yang, H., Shen, W., Xu, H., Hong, Z., & Liu, C. (2014, October). Prediction of the wind turbine performance by using BEM with airfoil data extracted from CFD. Renew Energy, 70, 107–115. https://doi.org/10.1016/J.RENENE.2014.05.002
- Zhang, M., Wu, Q., Wang, G., Huang, B., Fu, X., & Chen, J. (2020, May). The flow regime and hydrodynamic performance for a pitching hydrofoil. Renew Energy, 150, 412–427. https://doi.org/10.1016/J.RENENE.2020.01.006
- Zhu, H., Hao, W., Li, C., & Ding, Q. (2019, March). Numerical study of effect of solidity on vertical axis wind turbine with Gurney flap. Journal of Wind Engineering and Industrial Aerodynamics, 186, 17–31. https://doi.org/10.1016/J.JWEIA.2018.12.016