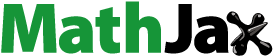
Abstract
A crucial factor in designing the wind turbine blade tips for improved aerodynamic performance is the winglet geometry. The potentials of curved planform winglet profiles for performance augmentation have not been adequately investigated. This study focuses on modifying the NREL Phase VI blade tip geometry with different winglet configurations using the S809 airfoil profile. The benchmark blade and the blade with a winglet were designed and simulated using CFD. Accordingly, three distinct winglet designs were created to characterize the flow behavior at the tip and tip vortices. For methodology design and validation, the NREL Phase VI blade served as the benchmark blade. Consequently, the CFD and QBlade simulation and numerical findings are compared with data from the NREL Phase VI experiment. When comparing the simulated parameters like power and pressure coefficients with measurements, there is an adequately high degree of agreement. Therefore, compared to the benchmark blade, the aerodynamic power shows a 5.34% to 9.97% gain, while the increase in axial thrust force shows 5% to 11.84% for the wind speed between 5 m/s and 13 m/s. Moreover, all the winglets show a comparable power boost at low wind speeds, while the blade with winglet (W2) marginally shows better aerodynamic performance at higher speeds. Furthermore, axial thrust force rises as the winglet curvature length increases, thereby winglet W3 shows a substantial axial thrust force rise. The results further demonstrate that the geometry of blade tip winglets can have a considerable impact on the wind turbine blade effectiveness as a whole.
1. Introduction
The strategies used currently to improve the efficiency of horizontal axis wind turbines involve the use of power curve remodeling devices, such as vortex generators, winglets, and serrated trailing edges, among others. Both the wing tip and the blade tip winglet curvatures or geometries are determined by a number of variables, including the cant angle, length, height, twist, sweep, toe, chord distribution, and also planform like rectangular, tapered, elliptical, etc., airfoil, and position, the wing suction or pressure side (Gertz et al., Citation2012; Guerrero et al., Citation2018; Khalafallah et al., Citation2019; Popescu et al., Citation2022). The curved blade’s shape effectively divides the high and low-pressure air, enhancing lift on the blade’s upstream side and decreasing induced drag brought on by the vortices. Likewise, vortex generators are a pair of tiny fins that are located close to the wind turbine blade’s root. They reduce airflow separation, which leads to a smoother flow across the blade. This increases the torque needed to turn the rotor and generate power with less turbulence.
The shape of the winglets and their direction upstream or downstream have an impact on the performance of turbine blades, according to several researchers who evaluated the tip vortex and characteristics of blades with winglets (Abdulkadir et al., Citation2015; Al-Abadi et al., Citation2018; Khalafallah et al., Citation2019; Mohammed et al., Citation2019). Other research (Guerrero et al., Citation2018; Gupta & Amano, Citation2012; Mohammed et al., Citation2019) examines the impact of blade winglet parameters on the effectiveness of wind turbine blades, specifically the modification of cant angle, sweep angle, dihedral angle, toe angle, winglet height impact, winglet curvature impact, taper ratio, and blended winglet. A study on the examination and blade tip winglets optimization utilizing the free wake vortex approach was conducted by Lawton and Crawford (Citation2014). Thus, it was determined that adding winglets may result in a 2% increase in wind power and a corresponding increase in thrust. A winglet that slightly reduces thrust while keeping power that is quite similar to that of a regular straight blade is also suggested.
A three-bladed horizontal axis wind turbine’s entire blade and bladelet configurations were designed utilizing a thorough 3D flow-field analysis in conjunction with multi-objective constrained shape design optimization, Reddy et al. (Citation2019). Results for a Pareto-optimized bladelet on a specific blade demonstrate that during off-design conditions, a more than 4% increase in the coefficient of power at a minimum thrust force penalty is feasible compared to the same wind turbine rotor blade without a bladelet. It is anticipated that even higher performance of a blade and bladelet combination will be possible whenever the blade and bladelet forms are concurrently optimized, if more complex bladelet shapes are taken into account, and if more robust response or reaction surfaces and support points are employed.
To determine the most effective stopping strategy of the induced flow at the tip, Al-Abadi et al. (Citation2018) conducted experimental evaluations of the turbulent free stream influence on the vortices of the tip created by blades of wind turbine and different winglet designs. The findings demonstrate that turbulence lowers tip losses by suppressing tip vortices. The results also encourage more research into the area surrounding the intersection of the near and far wakes to better comprehend how the exchange of energy and entrainment of the free stream support the recovery of the wake. The results of an investigation by Gaunaa and Johansen (Citation2007) into the theoretical issues and calculation outcomes pertaining to the usage of winglets on wind turbines revealed that winglets aligned downstream are superior to upstream ones in terms of optimizing the power coefficient and that the improvement in power output is not as great as what could be attained by simply extending the blade radially outward. Additionally, it is claimed that downwind winglets with shorter lengths (>2%) can achieve power coefficient increases comparable to those attained by radial wing extensions.
Variable cant angle winglets were suggested by Guerrero et al. (Citation2018) as a way to help aircraft achieve the optimum overall effectiveness, to reduce the induced drag due to the lift, at various values of attack angle. It is therefore recommended that for optimal performance and drag reduction, a cant angle of 15° be used. The use of winglets, according to Khalafallah et al. (Citation2019), can increase aerodynamic efficiency to the point where at the tip speed ratio of the design for downwind-swept blades with winglets facing upwind, the power coefficient increases by 4.39% with the winglet canting at 40° and twisting at 10°. Additionally, according to Abdulkadir et al. (Citation2015) study findings, a 26% lift-to-drag ratio increase is caused by the upwind winglet when compared to a conventional blade without a winglet, while the downstream winglet causes it to decrease by around 27%. It is also hypothesized that improved aerodynamic performance is significantly influenced by the wind turbine blade angle of the yaw. The best performance improvement, according to Mohammed et al. (Citation2019), was obtained when the length of winglets was kept at 6.32% of the wind turbine radius of the blade and canted at a 48.3° angle. The effect of winglet geometry and airfoil on wind turbine effectiveness was explored by Farhan et al. (Citation2018), who investigated and searched for the optimal design of winglet for the wind turbine blade. Using the model winglet design, it is predicted that the power generated by wind turbine will rise.
The winglets integration to improve the effectiveness of a wind turbine blade has been the subject of several studies, and the computational simulation was carried out employing the ANSYS Fluent CFD software and OpenFOAM. In addition, further research employing various designs of winglets with varying parameters like length, cant angle was computationally investigated as well as optimized employing computationally effective techniques; meta-heuristic algorithms like GA, PSO, ANN, etc. The SST k-ω turbulence model was widely used in investigations to simulate flow turbulence (Abdulkadir et al., Citation2015; Al-Abadi et al., Citation2018; Guerrero et al., Citation2018; Gupta & Amano, Citation2012; Khalafallah et al., Citation2019; Mohammed et al., Citation2019; Monier et al., Citation2014; Popescu et al., Citation2022; Reddy et al., Citation2019).
To predict aerodynamically the effectiveness of NREL Phase VI wind turbines, Monier et al. (Citation2014) solved steady-state RANS equations and applied the k-ε Launder-Sharma turbulence model. The results of computation and the experimental data have a good deal in common. It was suggested that the final optimized winglet produced more power by about 9%. Other studies investigated and centered on the impact of winglet use on the blade’s tip of an NREL Phase VI wind turbine, and how turbulence intensity affects a blade with and without a winglet. Geometry modeling and simulation are done using CFD. The computational conclusion produced by the modeling of (k-ω) SST turbulence is strongly supported by the NREL experimentally measured data between wind speeds of 5 m/s to 25 m/s (Farhan et al., Citation2018; Gupta & Amano, Citation2012; Mohammed et al., Citation2019; Monier et al., Citation2014; Shalini et al., Citation2021). Similar to this, Miguel Sumait et al. (Citation2020) used CFD to analyze the aerodynamics of a winglet with split geometry on a wind turbine blade by modifying the NREL Phase VI blade tip. According to the study, adding the winglet boosted power generation by 1.23% averagely in between 7 m/s and 15 m/s wind speed, as opposed to extra-long blades, split winglets with split geometry boosted it by 2.53%.
As per the literature review summary, winglets for wind turbine blades have not received as much attention as non-rotating blades. The literature revealed that several studies had been done on the impact of winglet parameters and configurations broadly for aircraft wings and moderately on the performance of wind turbine blades, as per Maughmer’s (Citation2003), but a winglet shape and airfoil profile effect for a wind turbine blade had not been thoroughly investigated. In addition, there is no detailed evidence about the potential of curved planform winglet shapes for performance augmentation. In particular, there has not been much research on or reported in the literature regarding the performance of curved planform winglet shapes with various winglet profiles. Moreover, the interaction between the winglet parameter and a wide variety of winglet geometry was not taken into account in earlier work on curved winglets. Considering the knowledge gap, the present study intends to evaluate the impact of curved planform winglet shape with S809 airfoil profile on the NREL Phase VI effectiveness. On account of the winglets’ curved design, they may have a substantial impact on dispersing wing tip vortices and reducing induced drag. Hence, three distinct winglet configurations were created and looked at to evaluate the flow behavior at the tip and tip vortices in this study. The NREL phase VI blade’s surrounding flow field with and without winglets was modeled using QBlade and CFD, and validation was completed by comparing the predicted power, coefficients of pressure, and thrust force with data from the experiment.
2. Blade geometry and methodology
The NREL Phase VI wind turbine blade was used to model and design the intended blade with a winglet. This blade, NREL Phase VI, underwent extensive experiments in the NASA Ames wind tunnel facilities (Hand et al., Citation2001). The experimental arrangements involve several sequences, for this study the sequence S was chosen. The open-source framework QBlade is created for the modeling, design, and optimization of wind turbines. It uses the BEM simulation method, and more recent upgrades have added the lifting line simulation method, which is a member of the vortex method family. The software has been completely integrated with the NREL FAST and Aerodyn modules for unsteady aerodynamics and aeroelastic modeling, as well as the XFOIL airfoil design and analysis tool. Each portion of the blade in QBlade is described by its position, chord, twist, airfoil, and related 360° polar. QBlade has complete documentation (database) for NREL Phase VI (Marten, Citation2016). Next, after designing the blade aerodynamically using QBlade software, the rotor BEM simulation module of QBlade allows us to analyze the blade performance, turbine definition, and simulation. The majority of winglet studies to date have concentrated on winglets with conventional planforms, leaving a substantial research gap on curved planform winglet profiles. Consequently, the effective cant angles for wind turbine winglets suggested by several studies are in the range of 30° to 60° (Elfarra et al., Citation2015; Gupta & Amano, Citation2012; Khalafallah et al., Citation2019; Mohammed et al., Citation2019). Accordingly, three curved planform winglet configurations with 30°, 45°, and 60° cant angles are designed; these winglets have crucial significance for the study that follows. The present study was intended to evaluate the impact of curved planform winglet shapes with S809 airfoil profiles on the NREL Phase VI effectiveness, with three distinct winglet cant angles and arc angles thereby different arc lengths. The NREL Phase VI benchmark blade had already been created in QBlade, initially; Table , and Figure ; ultimately, the benchmark blade and the winglet-incorporated blades from NREL Phase VI wind turbine blades were then combined to create a total of four different blade variants, Figures . The software ANSYS SpaceClaim was then used to design and create the winglets after the generated blade had been imported, Figure . Under this preliminary evaluation of the impact of curved planform, winglet configurations with fixed simple linear extension (50 mm), winglet lengths (70 mm), and different cant angles (30°, 45°, and 60°) were created and looked at to evaluate the flow behavior at the tip and tip vortices. Additionally, the cant angle and an arc angle are made the same (30°, 45°, and 60°); then the planform curvature radius and arc length of the winglet varied chord-wise in all three designs, and the S809 airfoil were used to generate the winglet, as shown in Table and Figure . Moreover, Figure shows the 2D view of the generated blade winglet sections which illustrate the winglet with the corresponding cant angle and other parameters.
Figure 2. The 2D section views winglet configurations with parameters (a) with cant and arc angle 30°; (b) with cant and arc angle 45°; (c) with cant and arc angle 60°; (d) isometric view.

Figure 3. Blade winglet configurations, (a) NREL Phase VI blade with winglet length 70.03 mm & cant angle 30° oriented to the suction side, (b) NREL Phase VI blade with winglet length 70.03 mm & cant angle 45° oriented to the suction side, (c) NREL Phase VI blade with winglet length 70.03 & cant angle 60° oriented to the suction side, (d) benchmark NREL Phase VI blade tip region without winglet.

Table 1. The NREL Phase VI rotor: geometry and design parameters (Hand et al., Citation2001)
Table 2. Design parameters and winglet configurations
The simulations and analysis were performed in terms of power improvement at 5 to 20 m/s wind speeds, and the sectional pressure coefficient distribution was also evaluated. The effects of various winglet configurations on the blade tip vortices, power output, and thrust force generation of the wind turbine blade were then investigated and finally, the better winglet configuration was selected.
2.1. Governing equations
The continuity, momentum, and energy equations are the fundamental governing equations of fluid dynamics and serve as the cornerstone of computational fluid dynamics because all CFD is entirely based on these equations (Tu et al., Citation2013). An important application of computational aerodynamics is the aerodynamics of airfoil profiles. Reynolds-Averaged Navier–Stokes equations (RANS) in conjunction with different turbulence models are frequently used to simulate flow field around airfoil profiles (Schaffarczyk, Citation2014).
2.1.1. The continuity equation
2.1.2. The momentum equations
As an alternative, the flow equations (transport equations), which are essential to the finite volume CFD approach (Moukalled et al., Citation2016), can be expressed in integral forms:
for time-dependent processes (unsteady processes); where general flow variable,
is the diffusion coefficient and
the source term.
2.2. Computational domain and mesh generation
The simulations were carried out using FLUENT, a computational fluid dynamics program. Moreover, for this simulation, the RANS Equations with the chosen turbulence model, (k-ω) SST model was used. According to findings in the literature, the (k-ω) SST turbulence model maintains an acceptable balance between accuracy and computational effort. The (k-ω) SST model was chosen because turbulent flow over airfoils has been effectively simulated using it. Moreover, the (k-ω) SST is better than other two-equation turbulence models for external flow simulation, but typically not sufficiently precise to be used as a reference for the exact numerical prediction of aerodynamic drag and lift forces (Lawson et al., Citation2011; Popescu et al., Citation2022; Zhang et al., Citation2023). The rotational axis is counterclockwise about the z-axis because the computational domain employs a Cartesian coordinate system, where the positive x-axis is in the direction of the blade span, the negative y-axis is in the vertical direction, and the positive z-axis is in the direction of the stream.
The boundary conditions were defined by modeling explicitly one turbine blade during computational simulation; it explains the two NREL Phase VI turbine blades’ 180° rotation. The periodic boundary condition was used for the second turbine blade. A simulation was performed using the moving reference frame (MRF) which considers the blade’s rotating motion in the domain. Investigations were carried out at 72 rpm angular speed. Moreover, for the inlet boundary, 5 m/s to 20 m/s wind speed values were considered, and for the outlet boundary, a zero Pascal (gauge) pressure was chosen. The turbine blade surface was assigned the no-slip condition. Since the rotational flow field simulation is based on the MRF function, a cylindrical shape of a computational domain is designated which has been made equal to 20 m and 50 m from the blade in the upstream and downstream directions, respectively. Additionally, the circular section of the computational domain, the symmetry boundary, is chosen as a cylinder shape and its radial values at the inlet and outlet boundaries are set to 6R and 7R, respectively (where R is the turbine blade’s radius). Generally, symmetry boundary conditions are employed when there is mirror symmetry between the desired flow solution pattern and the physical geometry of interest. The proximity and curvature sizing function was applied to mesh the model for refinements, and 13 inflation layers were used so that the first layer height was kept at m which keeps y+ values below 5. Additionally, a rectangular local refinement region around the blade was implemented. Furthermore, to ensure accurate simulation the leading and trailing edge regions of the blade are enabled to have well-refined meshes. The volume mesh was then meshed using poly-hexcore meshing. A poly-hexcore meshes generally, the first application of Mosaic technology, results in a lower mesh count, relative to polyhedral, thereby reducing the simulation time. The resulting mesh consists of the number of cells 1.79 million, faces number 7.70 million, and nodes number 4.43 million, with an average 0.9 orthogonality quality and a 0.6 maximum skewness. Since utilizing a transient solver did not significantly deviate from the steady-state solver result, a pressure-based steady-state RANS solver was employed to shorten calculation times. A report definition including the moment, force and residual on the rotor blade was considered and residual values below 10−4 were ensured for convergence. The resulting domain mesh and the mesh near the blade are shown in Figure .
Figure 4. (a) Physical domain, (b) a rectangular local refinement region around the blade (c) a poly-hexcore domain mesh, (d) blade surface mesh.

Taking torque and thrust force as the response parameters, the simulation results are checked for grid independence using mesh refinement. As revealed in Table , torque and thrust force became stable and the deviation is less than 1% when cell numbers are greater than 1.5 million cells. As a result, a total of 1.67 million cell count is selected subsequently.
Table 3. Mesh independence test
3. Results and discussion
The established approach and the simulation results were validated using the experimental data from the NREL Phase VI wind turbine. Therefore, the simulation results were divided into two categories. The first is the validation part which is intended to validate and assess the computational model’s capability to predict the NREL Phase VI experimental data. The second is the CFD simulation results that were obtained by the modeled and designed blade winglets. The operating conditions for the experiment varied from 5 to 20m/s wind speed. The generated simulation results were compared, interpreted, and analyzed for five sectional positions of the blade span-wise at test wind speeds between 5m/s and 20m/s; the five span-wise locations of the blade considered are 30%, 47%, 63%, 80%, and 95% of the blade span and similarly, the comparison parameters are (i) the pressure coefficient distribution in all section five section of the blade for selected wind speeds; (ii) wind turbine aerodynamic power, the global torque and thrust force; (iii) the streamline flow distribution on the blade surface, the distribution of axial velocity and vorticity. The NREL Phase VI baseline (benchmark blade) simulation was conducted at wind speeds of 5–10, 13, 15, and 20 m/s, while the NREL Phase VI blade with winglet was simulated at wind speed of 5 to 11 m/s. The tip-speed ratio of 1.5 to 7.5 is considered which corresponds to the angular speed of 72 rpm; so the design tip-speed ratio is 5.2 which correspond to a design wind speed of 7.2 m/s and blade radius of 5.029 m. In particular, as shown in Figure , these CFD simulation findings were contrasted with data from the NREL Phase VI turbine blade experiment and related works published in the literature.
The equation used to compute the pressure coefficient is (Guerrero et al., Citation2018; Popescu et al., Citation2022; Zhang et al., Citation2023):
where i stands for the ith blade portion, p denotes local static pressure, pref indicates a reference, the free stream static pressure at the domain inlet in this instance, denotes air density,
denotes inlet velocity,
denotes rotor rotational velocity, and ri denotes ith section radial position.
The wind turbine aerodynamic power is calculated by
where P is the computed aerodynamic power (W); is integrated torque (Nm);
is the rotor angular speed (rad/s).
3.1. Validation of NREL phase VI CFD simulation
The simulation results are illustrated by comparing the aerodynamic power at different wind speeds as well as the chord-wise pressure coefficient distributions at different blade sections-span-wise; Figure depicts the comparison between the simulated power and the sequence S experimental data of NREL Phase VI with a good agreement between the simulated power and data from the experiment. Further, the simulated results of the baseline blade show that the CFD power prediction is within ± 3% of the experimental results, while the QBlade power prediction is within ± 11.69%.
Furthermore, related works in the literature showed that wind turbine blade winglet performance can be reasonably predicted using the CFD simulation. Therefore, as studied by (Elfarra et al., Citation2015; Farhan et al., Citation2018; Miguel Sumait et al., Citation2020; Shalini et al., Citation2021), the NREL Phase VI wind turbine blade tip modification and winglet incorporation under various operating conditions can be designed and predicted accurately using CFD. Many of these computational studies encountered numerous problems at higher wind speeds, where stall dominates the flow, with an escalating tendency for divergence from the experimental data and disparities, Figure . In our view and from literature review, the less successful attempts can be primarily due to inadequate (low quality) meshing, poor model selection for turbulence, or other types of user errors. Some studies also experienced mesh density issues, which were likely brought on by a lack of sufficient computational capacity (Madsen et al., Citation2022; Popescu et al., Citation2022; Zhang et al., Citation2023).
Likewise, the comparisons of measured and simulated pressure coefficients for different wind speeds can be illustrated at the blade span-wise location of 30%, 47%, 63%, 80%, and 95%. EquationEquation 88
8 is used to calculate the pressure coefficients at this span-wise location of the blade; a robust agreement exists between predicted and data from the experiment at all span-wise sections for the pressure coefficient distributions of 7 m/s, and 9 m/s wind speeds where the prevailing flow is dominated by attachment and minor stall occurrences manifested at these wind speeds as depicted in Figures . However, the deviation between the experimental data and simulated pressure coefficient distributions is noticeable at this speed as well as at higher wind speeds, particularly in the root region, for instance, 30% inboard span of the blade. This discrepancy is attributed to a strong stall and the transition flow that occurred at this speed in this region; likewise, at higher wind speeds this phenomenon occurs including flow separation. Literature indicates similar results have been obtained by several studies (Elfarra et al., Citation2015; Farhan et al., Citation2018; Miguel Sumait et al., Citation2020).
Contours of relative velocity with streamlines help examine flow separation at different span-wise locations of the blade. As a result, some plots are produced at wind speeds between 7 and 9 m/s. Minor separation seems to occur at 7 m/s wind speed as observed from Figure , that is, an attached flow and this is the reason that the pressure distribution results agreed well with data from the experiment at lower speeds of the wind. But, at 9 m/s wind speed, a small separation occurs as depicted in Figure . The flow separation at this wind speed shows an increasing trend from the low intensity at the 30% inboard section then attaining its high intensity at the sections of 47% to 63% then decreases at the section of 80%, and lastly diminished to a minor separation at the section of 95% of the blade. Moreover, at 13 and 15 m/s, the flow separation and vortices formation occurred at all sections of the blade. Similarly, Figure shows an expanded view of the flow separation at 13 and 15 m/s for 95% of the span. Due to this, the simulated pressure distributions deviate more from the experimental data at these speeds.
3.2. CFD simulation results of winglet configurations and analysis
Under this scenario, the winglet profiles were constructed using the S809 airfoil profile which has the same aerodynamic characteristics as the benchmark blade tip region airfoil. The predicted results of the benchmark blade and blade with winglets are compared based on wind turbine blade aerodynamic power and axial thrust force, as shown in Figures . There is a substantial power increment gained by the blade with winglets but the blade with W3 winglet slightly showed more thrust force in addition to gain in aerodynamic power under the considered wind speed of 5 to 13 m/s. Wind turbine blade aerodynamic power increased on average, across the simulated 5 to 13 m/s wind speeds, by 5.34% to 9.97% when comparing the winglet integrated blade to the benchmark blade. Moreover, the results revealed that the blade with the W1 and W2 winglet showed a 5.37% to 9.97% improvement in power generation at the same time showed a 5% to 10.54% increment in axial thrust force at wind speed 5 to 13 m/s and outperformed as compared to the W3 winglet configuration which showed 5% to 11.84% increment in the axial thrust force at the same wind speed range. Furthermore, the results show that in the range of wind speed 5 to 13 m/s, blades with winglets W1 and W2 angles have minor differences. However, the blade with the W1 shows slightly better performance at wind speeds smaller than 9 m/s while W2 marginally shows good performance at wind speeds above 7 m/s. This performance improvement is the result of pressure coefficient improvement in addition to the reduction in the tip vortices and induced drag.
Figure 11. Comparison of the CFD simulated power for the benchmark without winglet and blade with variant winglet configurations.

Figure 12. Comparison of the CFD simulated thrust force for the benchmark without winglet and blade with variant winglet configurations.

Generally, blade winglets in addition to their performance improvement also increase thrust force which is not desirable. Accordingly, a thrust force increment by 5% to 11.48% in ranges of wind speed 5 m/s to 13 m/s is observed. This increase in thrust force requires additional consideration of a comprehensive structural system that can support the added loads.
On an operational wind turbine blade, the subsequent pressure differential results in flow inward along the suction side of the blade and outward along the pressure side towards the tip, so induced drag is created at the trailing edge where vorticity is created. The winglet’s primary function is to dilute and disperse the vortices of the tip, thereby lowering the induced drag. In order to analyze the vortices formed around the tip region of the blade, a ZX-plane that cuts the blade right at the tip of the trailing edge was used, the right-hand side illustrates the sliced portion in the enlarged view which shows the blade’s trailing edge section at (y = −0.17) which is located at 96% of the benchmark tip chord from the leading edge, as shown in Figure . This allows the vortices to be observed using vorticity and velocity vector field representation, as illustrated in Figures for 9 m/s wind speed.
Figure 13. ZX-plane that cuts the blade right at the tip of the trailing edge and the sliced portion an enlarged view.

The results are essentially different for the three blades when the vortices and velocity magnitude of the benchmark blade and blade with the winglet are compared, showing that the blade with the winglet has fewer vortices than the benchmark blade. This is the result of adding winglets to the blade, which, in comparison to the base blade, lowers the swirling motion of the air around the tip. Additionally, as illustrated in Figures , the span-wise flow and vortices at the blade tip are significantly reduced by all blades with winglets. Additional information regarding the vortex center and rolling direction is depicted in Figures . Although the air is still swirling in all scenarios, the vortex core distance from the blade surface is quite different so that the benchmark blade’s vortex core is the closest to the blade surface, while the blades with winglet have both wide and far vortex core, as shown in Figures .
Figures display the extracted distributions of axial velocity and vorticity along the z-axis from r/R = 95% of the span to the tip and perpendicular to the plane of rotation in order to allow for more quantitative comparisons. The distributions demonstrate the impact of adding a winglet, particularly in the area near the tip, as illustrated in Figures . The axial velocity reaches the value of the free-stream velocity when it is far from the tip vortex areas. Likewise, the vorticity is greatest at the center of the vortex (place of largest velocity drop) and approaches zero outside of it. The vorticity distributions shown in Figure demonstrate that, in the winglet situation, the levels of vorticity within the vortex cores are considerably decreased, signifying the declining strength of the tip vortices.
Figure 15. Velocity magnitude, at 9 m/s around tip region: benchmark (left) and blade with winglet W2 (right).

Furthermore, as demonstrated in Figures the blade with winglets has a reasonably strong impact on the axial velocity distribution and vorticity magnitude at the blade tip.
Figure 16. The distribution of axial velocity obtained from the span-wise position r/R = 0.95 to the tip both for the benchmark and winglet cases, at 9m/s.

Figure 17. The vorticity distributions obtained from the span-wise position r/R = 0.95 to the tip for the benchmark and winglet cases, at 9 m/s.

The contours of surface pressure on the blades show that the local pressure distribution across the blade’s surface are essentially similar among the blades. However, a considerable variation in magnitude and intensity can be seen around particular locations, so these noticeable differences are observed at the blade tip region, Figure . Moreover, Figure shows the local static pressure contour at the entire blade surface and tip region. As can be seen, the benchmark, and blades with winglets pressure contours around the tip region are fundamentally different. Consequently, the location of the vortex core at the blade tip is illustrated by the pressure contour and highlights it with a dark blue color. This makes it easy to see the tip vortex. As a result, the blades with winglets effectively lower the tip vortex effect by displacing the vortex’s center farther from the blade’s tip, while from the benchmark blade tip region the vortex core does not move away farther.
The result of the winglet’s addition so clearly demonstrated that it has a considerable impact on the distribution and size of the low-pressure region both on the suction and pressure side. Additionally, compared to the benchmark blade, the blade with winglet has a less intense low-pressure area on the pressure side and a high-pressure area with greater intensity and magnitude at the leading edge; however, on the suction side, the pressure contours of the winglets resembles the benchmark except for a much smaller low-pressure area close to the leading edge, Figure . Consequently, as shown in Figure , low-pressure zone that varies depending on the configuration is produced on the suction side near the blade tip, boosting the pressure gradient across the rotor and causing an increase in thrust.
Figure 19. Benchmark and blade with winglets: surface local pressure on the suction (a) and pressure side (b) at section 98% of the span (r = 5.1274) and wind speed of 7m/s.

To realize the effect of the winglet configurations in detail, the pressure coefficients close to the blade tip, first with respect to the benchmark blade at section r = 5.1274 m, i.e., r/R = 98% of benchmark blade, the benchmark, W1, W2, and W3 winglets were examined, as shown in Figure . Inspection of the results show that substantial pressure difference on the suction side but a negligible variance in pressure on the pressure side exists, except for winglet W3 at the trailing edges where the pressure difference is marginally significant. Similarly, at section r = 5.2724 m, i.e., r/R = 98% of the blade with winglet section then W1, W2, and W3 winglet configuration influences were compared. Figure shows that, at section r = 5.2724 m, blades with winglet W1 and W2 configurations followed the same pattern and reveal slight significant pressure difference on the suction side. However, the difference in pressure on the pressure side shows negligible variation. While examining the effect of winglet W3, the pressure differences on both the suction and pressure side deviate marginally from W1 and W2. These variations are predominantly attributed to the effect of the vortices distribution at the blade tip, as can be verified from Figures . The pressure coefficient plots in Figure at a wind speed of 9 m/s particularly show that the blade with winglets demonstrates that the pressure coefficient distributions are enhanced more on the span-wise suction side, especially on the regions close to the blade tip. In both cases, the cumulative improvement is greater in r/R = 98% span-wise regions of the blade with W2. Therefore, the improvement in pressure coefficients shows that the use of winglets causes the blade to extract greater energy from the fluid flow.
4. Conclusions and future works
The aerodynamic performance of wind turbine blades can be improved through blade tip modification with effective winglet configurations. Consequently, winglets have the potential to dilute and disperse the vortices of the tip, thereby lowering the induced drag. The purpose of the study was to determine whether tip modification and incorporating various winglet configurations having the S809 airfoil profile and varying curvature would enhance the NREL Phase VI blade’s aerodynamic performance. Accordingly, three winglet configurations with fixed winglet lengths of 0.07 m and variable curvature having cant and arc angles of 30°, 45°, and 60° are designed and created. The findings show that the inclusion of winglets causes observable increases in torque and axial thrust force. As a result, power generation is improved in the range of wind speeds from 5 to 13 m/s by an average of 5.37% to 9.97% using winglets that bend toward the suction side. Furthermore, with the winglet cant and arc angle is 45° (W2) and fixed winglet length of 0.07 m, the performance is improved marginally better than others at wind speed above 7 m/s. Moreover, the axial thrust force rises as the winglet curvature length increases, thereby winglet W3 shows a substantial axial thrust force increment. Additionally, all winglets W1, W2, and W3 show a comparable power boost at low wind speeds of 5 and 7 m/s (9.53%, 7.09%), (9.52%, 7.64%), and (9.84%, 7.54%), respectively, compared to the benchmark blade. Furthermore, the planform, airfoil profiles of a winglet as well as its shape have a big impact on the axial thrust force and effectiveness of the wind turbine blade. In conclusion, this suggests that a winglet has potential benefits for the refurbishment of existing blades for a power boost and for improving a wind turbine blade’s effectiveness when the axial thrust force meets the required constraints. In conclusion, as opposed to a straight extension of the tip along the span-wise location, it is certainly advantageous to curve the tip to form a winglet shape of different variants.
The subsequent work and effort will be aimed at incorporating additional parameters and a wide range of winglet configurations because the current paper only includes a small number of winglet parameters and configurations. Furthermore, a multi-objective optimization will also be considered to take into account the impact of other winglet design parameters and operating conditions.
Acknowledgments
Partial support from Jimma Institute of Technology Center of Excellence is gratefully acknowledged.
Disclosure statement
No potential conflict of interest was reported by the author(s).
References
- Abdulkadir, A., Chowdhury, H., Bavin, L., & Firoz, A. (2015). An aerodynamic study of a domestic scale horizontal axis wind turbine with varied tip configurations. Procedia Engineering, 105, 757–21. https://doi.org/10.1016/j.proeng.2015.05.067
- Al-Abadi, A., Kim, Y., Ertunc, Ö., Epple, P., & Delgado, A. (2018). Interaction between free-stream turbulence and tip-vortices of wind turbine blades with and without winglets. Journal of Physics Conference Series, 1037(7), 072025. https://doi.org/10.1088/1742-6596/1037/7/072025
- Elfarra, M. A., Sezer Uzol, N., & Sinan Akmandor, İ. (2015). Investigations on blade tip tilting for hawt rotor blades using CFD. International Journal of Green Energy, 12(2), 125–138. https://doi.org/10.1080/15435075.2014.889007
- Farhan, A., Hassanpour, A., Burns, A., & Motlagh, Y. G. (2018). Numerical study of effect of winglet planform and airfoil on a horizontal axis wind turbine performance. Renewable Energy, 131, 1255–1273. https://doi.org/10.1016/j.renene.2018.08.017
- Gaunaa, M., & Johansen, J. (2007). Determination of the maximum aerodynamic efficiency of wind turbine rotors with winglets. Journal of Physics Conference Series, 75, 012006. https://doi.org/10.1088/1742-6596/75/1/012006
- Gertz, D., Johnson, D. A., & Swytink-Binnema, N. (2012). An evaluation testbed for wind turbine blade tip designs — winglet results. Wind Engineering, 36(4), 389–410. https://doi.org/10.1260/0309-524X.36.4.389
- Guerrero, J., Sanguineti, M., & Wittkowski, K. (2018). CFD study of the impact of variable cant angle winglets on total drag reduction. Aerospace, 5(4), 126. https://doi.org/10.3390/aerospace5040126
- Gupta, A., & Amano, R. S. (2012). CFD analysis of wind turbine blade with winglets, Proceedings of the ASME. https://doi.org/10.1115/DETC2012-70679
- Hand, M., Simms, D., Fingersh, L., Jager, D., Cotrell, J., Schreck, S., & Larwood, S. (2001). Unsteady aerodynamics experiment Phase VI: Wind tunnel test configurations and available data campaigns. NREL/TP, 500–29955. https://doi.org/10.2172/15000240
- Khalafallah, M. G., Ahmed, A. M., & Emam, M. K. (2019). The effect of using winglets to enhance the performance of swept blades of a horizontal axis wind turbine. Recent trends in fluid dynamics. Advances in Mechanical Engineering, 11(9), 168781401987831. https://doi.org/10.1177/1687814019878312
- Lawson, M. J., Li, Y., & Sale, D. C. (2011). Development and verification of a computational fluid dynamics model of a horizontal-axis tidal current turbine. National Renewable Energy Laboratory. https://doi.org/10.1115/OMAE2011-49863
- Lawton, S., & Crawford, C. (2014). Investigation and optimization of blade tip winglets using an implicit free wake vortex method. Journal of Physics Conference Series, 524, 012033. https://doi.org/10.1088/1742-6596/524/1/012033
- Madsen,M. H. A., Zahle, F., Horcas, S. G., Barlas, T. K., & Sørensen, N. N. (2022). CFD-based curved tip shape design for wind turbine blades. Wind Energy Science, 7(4), 1471–1501. https://doi.org/10.5194/wes-7-1471-2022
- Marten, D. (2016). QBlade v0.95, guidelines for lifting line free vortex wake simulations. https://doi.org/10.13140/RG.2.1.1663.1929
- Maughmer, M. D. (2003). Design of winglets for high-performance sailplanes. Journal of Aircraft, 40(6), 1099–1106. https://doi.org/10.2514/2.7220
- Miguel Sumait, S., Binoe Eugenio, A., & Louis, A. M. D. (2020). Aerodynamic Investigation of a Horizontal Axis Wind Turbine with Split Winglet Using Computational Fluid Dynamics. Energies, 13(18), 4983. https://doi.org/10.3390/en13184983
- Mohammed, K., Ibrahim, M. M., Abdel Hamed, H. E., & Ahmed, F. (2019). AbdelGwad: Investigation of a small horizontal axis wind turbine performance with and without winglet. Energy, 187, 115921. https://doi.org/10.1016/j.energy.2019.115921
- Monier, A., Elfarra, N. S.-U., & Akmandor, I. S. (2014). NREL VI rotor blade: Numerical investigation and winglet design and optimization using CFD. Wind Energy, 17(4), 605–626. https://doi.org/10.1002/we.1593
- Moukalled, F., Mangani, L., & Darwish, M. (2016). The finite volume method in computational fluid dynamics, an advanced Introduction with OpenFOAM and Matlab. Springer. https://doi.org/10.1007/978-3-319-16874-6
- Popescu, F., Mahu, R., Rusu, E., & Ion, I. V. A. (2022). Robust and efficient computational fluid dynamics approach for the prediction of horizontal-axis wind turbine performance. Journal of Marine Science and Engineering, 10(9), 1243. https://doi.org/10.3390/jmse10091243
- Reddy, S. R., Dulikravich, G. S., Sobieczky, H., & Gonzalez, M. (2019). Bladelets—winglets on blades of wind turbines: A multiobjective design optimization study. Journal of Solar Energy Engineering, 141(6), 061003. https://doi.org/10.1115/1.4043657
- Schaffarczyk, A. P. (2014). Introduction to wind turbine aerodynamics. Green energy and technology. Berlin Heidelberg: Springer-Verlog. https://doi.org/10.1007/978-3-030-41028-5
- Shalini, V., Akshoy Ranjan, P., Anuj, J., & Firoz, A. (2021). Numerical investigation of stall characteristics for winglet blade of a horizontal axis wind turbine. E3S Web of Conferences, 321, 03004. https://doi.org/10.1051/e3sconf/202132103004
- Tu, J., Yeoh, G. H., & Chaoqun, L. (2013). Computational fluid dynamics: A practical approach (2nd ed.). Elsevier. https://doi.org/10.1016/C2015-0-06135-4
- Zhang, Z., Limin, K., Zhaolong, H., Dai, Z., Yongsheng, Z., Yan, B., Lei, D., Jiahuang, T., Yaoran, C., & Mingsheng, C. (2023). Comparative analysis of bent and basic winglets on performance improvement of horizontal axis wind turbines. Energy, 281, 128252. https://doi.org/10.1016/j.energy.2023.128252