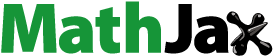
Abstract
In this article, we report the design and fabrication of a ‘do-it-yourself’ microfluidic channel with a circular cross-section, created outside a cleanroom facility. In this approach, the fabrication of the channel do not require any chemical etching or lithography steps, making it safe and suitable for small research groups. The channel is fabricated using the soft-lithography method in polydimethylsiloxane (Sylgard® 184). A copper wire with a diameter of 240 µm is used as a mold, and the channel is created using the wire pull-out method. This work also addresses the optimal use of polydimethylsiloxane in channel fabrication, as well as the challenges faced when connecting the microchannel to the outside world via tubing and techniques to overcome them. All simulations were conducted using COMSOL Multiphysics 6.0. The analytical, simulation, and test results, show a good match.
Reviewing Editor:
1. Introduction
Benefits such as high throughput, reduced analysis time, portability, minimal use of samples and reagents, and low cost make microfluidic devices attractive for a wide variety of applications. These include on-chip mixing of two or more fluids, Polymerase Chain Reaction (PCR), separation of plasma from whole blood, and many more. These microfluidic chips are also known as Lab on a Chip (LOC) or micro Total Analysis System (µTAS) (Amirifar et al., Citation2022; Hassanpour-Tamrin et al., Citation2021; Liu, Citation2012; Oliveira et al., Citation2019; Preetam et al., Citation2022; Sackmann et al., Citation2014; Shepherd et al., Citation2021; Tiwari et al., Citation2020). These devices can not only be used at the patient’s location, but also provide real-time test results. This can be useful for early disease detection, timely intervention by medical experts, potentially leading to significant savings in medical expenses (Eftekhari et al., Citation2021; Paiè et al., Citation2018; Qi et al., Citation2021; Wang et al., Citation2018; Yin et al., Citation2022).
Due to the broad range of applications, many researchers are working towards the design and fabrication of low-cost microfluidic structures. Gautam et al. (Citation2018) fabricated a microfluidic chip for Bulk Acoustic Wave (BAW)-based manipulation of cells and microparticles. An aluminum plate with a thickness of 5 mm was used as the substrate material. The channel pattern was designed using AutoCAD, and a channel with a depth of 80 μm was milled into the aluminum substrate. A thin layer of polydimethylsiloxane (PDMS) was prepared by spin-coating an uncured PDMS solution on a Mylar plastic sheet, followed by curing at 65 °C for 15–30 minutes. The cleaned aluminum plate with the channel pattern and the cured PDMS layers were exposed to air plasma for 30 seconds and then bonded together to create the functional channel. Inlets and outlets were created using Harris Uni-Core punches, and tubing was connected to interface the channel with the external world.
Razavi Bazaz et al. (Citation2020) reported the fabrication of microfluidic channels with various cross-sections using an additive manufacturing technique. The process begins with designing the channel model using a CAD tool, followed by printing the model using a high-resolution DLP/SLA 3D printer. The 3D printed model was attached using double-sided transparent tape (ARcare, Adhesive Research) to a PMMA sheet, and the entire structure was pressed with tweezers until all trapped air bubbles were removed. The major limitations of this approach are the need for a high-resolution 3D printer and the surface roughness of both the double-sided tape and the 3D printed parts.
Tiwari et al. (Citation2020) described the fabrication of a low-cost microfluidic channel using soft lithography. To do this, they started by designing the channel pattern using L-edit. They then printed this pattern onto Photopaper. Next, they transferred the pattern from the paper to a PCB plate using a hot iron box. The unwanted copper layer from the plate was etched using ferric chloride solution. Once the master was ready, it was cleaned. Next, the PDMS and bonder mixture were poured onto the master, followed by degassing to remove air bubbles from the PDMS mixture. Once the PDMS was devoid of air bubbles, it was placed on a hot plate (95 °C for 20 minutes) to cure the PDMS layer. Finally, the cured PDMS layer was bonded with a glass plate using oxygen plasma treatment. However, there were limitations, such as the copper layer’s thickness affecting the channel’s height, and the use of expensive oxygen plasma equipment to bond the glass plate with the PDMS.
Kim et al. (Citation2018) reported on the fabrication of a microfluidic channel using a femtosecond laser. The process began by directly writing a microchannel pattern onto a fused silica glass substrate with dimensions of 25 mm × 25 mm × 1 mm. Holes for the inlet and outlet were created, followed by etching with 20% HF acid for 30 minutes to achieve the microchannel pattern and clear inlet/outlet holes. Subsequently, DI water and piranha cleaning were performed to attain a highly hydrophobic surface for an improved laser welding process. Finally, a glass substrate was placed on top of the patterned glass substrate, and direct bonding was achieved using the femtosecond laser. The channel exhibited excellent bonding strength of 7.5 MPa (glass to glass), while tubing connections could withstand pressures of up to 1.1 MPa and 1.4 MPa for two different samples. The requirement for specialized equipment makes device fabrication costly, and channel roughness remains a major concern in femtosecond laser-based channel fabrication.
presents a comparison of different techniques for creating microfluidic channels. Looking at , it’s evident that the femtosecond laser-based process (Kim et al., Citation2018) is the fastest, but it raises the device cost due to the need for advanced equipment. Additionally, achieving a smooth channel surface is challenging. The additive manufacturing technique (Razavi Bazaz et al., Citation2020) offers the smallest features and can produce microfluidic devices in less than 2 hours, but it suffers from high channel roughness. The approach by Gautam et al. (Citation2018) involves CNC-based milling, which might lead to elevated channel roughness and longer fabrication times. Tiwari et al. (Citation2020) fabrication technique has a drawback of poor minimum feature size.
Table 1. Comparison of various channel fabrication techniques.
Song et al. (Citation2010) reported the fabrication of a circular microfluidic channel using a wire removal technique. To create the channel, they used a 300 µm diameter solder wire, which was bent into various shapes to form the mold for the microfluidic structure. Once the mold was ready, a mixture of PDMS base and bonder in a 9:1 ratio was poured onto the mold and degassed, followed by curing the PDMS on a hotplate at 100 °C for 1 hour. After curing of PDMS, it was placed in a vacuum oven at 190 °C. When the solder wire melted, it was removed from the channel, and finally, the inner wall of the channel was coated with a thin layer of PDMS to repair any imperfections caused during wire removal. There are a few major issues with the approach reported by Song et al. first, traces of solder wire inside the channel may affect its suitability for biomedical analysis. Second, coating the inner wall of the channel may alter its diameter. Third, positioning the mold during PDMS curing must be carefully managed so that the solder wire does not come too close to the surface of the PDMS layer (Song et al., Citation2010). Li and Xu (Citation2015) reported the fabrication of various microfluidic channel shapes based on a similar idea presented by Song et al. They used tungsten wires with diameters ranging from 9 µm to 100 µm as molds for the channels. A mixture of PDMS base and bonder was used in a 10:1 ratio, and curing was performed at 70 °C for 1 hour. Once the curing was complete, the template wire was slowly removed mechanically. If the channel structure was complex, and difficult to remove the template wire, ethanol could be used as a lubricant. As PDMS swells in ethanol, it makes it easier to remove the tungsten wire (Li & Xu, Citation2015). Effati and Pourabbs (Effati & Pourabbas, Citation2018) also reported the design and fabrication of microchannels using the wire removal technique for droplet generation. Both metallic (NiCr wire) and nylon (3m3 fishing line) threads were used as template (mold) for the microfluidic structures. The diameters of the template wires ranged from 150 to 800µm. A mixture of PDMS base and binder in a 10:1 ratio was poured onto the template, and curing was performed at 100 °C for 35 minutes. A mixture of chloroform and diisopropylamine (70/30 v/v) served as a lubricant for easy removal of the template wire. Moreover, the fabrication method of microchannels reported by Effati and Pourabbs required a significantly smaller amount of PDMS (Effati & Pourabbas, Citation2018). Rathod et al. (Citation2017) reported the fabrication of circular microchannels using the wire removal technique for culturing Human Umbilical Vein Endothelial Cells (HUVECs). The diameters of the templates used ranged from 120 µm to 450 µm. Rathod et al. employed a blend of PDMS Sylgard 527 and Sylgard 184 in various weight ratios to achieve microchannels with varying rigidity along the length of the channel (Rathod et al., Citation2017).
lists the comparison of various fabrication approaches to realize circular microfluidic channels in PDMS material and possible channel diameters. It can be seen from that positive-photoresist reflow and PDMS coating-based fabrication can only be carried out in cleanroom facilities, which is a costly approach, and researchers from small organizations do not have access to such facilities. Hence, it is not selected for device fabrication. It can also be observed from that the wire-removal technique can fabricate channels with diameter ranges from a few micrometers to several hundred micrometers, and it does not require a reverse molding process to prepare the template. The single use of a mold is the only limitation of this fabrication process.
Table 2. Comparison of circular microchannel fabrication methods.
Due to the simplicity in fabrication (Gautam et al., Citation2018; Kim et al., Citation2018; Razavi Bazaz et al., Citation2020; Tiwari et al., Citation2020) and compatibility with photolithography techniques, rectangular microfluidic channels have been extensively researched. Moreover, mathematical simulation of fluid flow and heat transfer within rectangular channels is less complicated when compared to complex channel designs. The broad range of applications for microfluidic devices has attracted numerous researchers to create cost-effective approaches to address a variety of challenges. Consequently, there exists substantial untapped potential for alternative geometries with cross-sectional shapes that are challenging to create using conventional microfabrication methods. The main objective of this work is to fabricate Do-It-Yourself (DIY) microchannel with minimal available facilities. Therefore, the wire-removal technique is used for device fabrication. The fabrication technique reported in this article requires a minimum amount of PDMS.
2. Governing equations
Fluid flow in the circular channel can be categorized based on the Reynolds number. If the Reynolds number is below 2300, flow is laminar; from 2300 to 4000, flow is transitional; and above 4000, the flow is turbulent. The Reynolds number is given as (Yunus, Citation2010)
(1)
(1)
Where ρ is the density of the fluid, obtained from material properties, Vavg is the average velocity, D is the diameter of the circular microfluidic channel, and µ is the dynamic viscosity of the fluid. EquationEquation (1)(1)
(1) can be rearranged to find the average velocity (Yunus, Citation2010)
(2)
(2)
The hydraulic diameter of the circular channel is given as (Yunus, Citation2010)
(3)
(3)
Where AC is the cross-sectional area, and r is the radius of the channel. Substituting in EquationEq. (3)
(3)
(3) , it simplifies to
(4)
(4)
From EquationEq. (4)(4)
(4) , it can be observed that the hydraulic diameter is the same as the diameter of the circular channel.
The average velocity, Vavg, remains constant in incompressible flow for a fixed channel diameter. If the mass flow rate or the volumetric flow rate is known, the average velocity can be easily calculated. The mass flow rate in a circular channel is given as (Yunus, Citation2010)
(5)
(5)
The volumetric flow rate in a circular channel is given as (Yunus, Citation2010)
(6)
(6)
Vmax is the maximum velocity of fluid at the centre of the channel, and it is approximately twice Vavg for the circular microchannel. The residence time of the fluid in the microchannel, with length L, is given as (Kandlikar et al., Citation2005)
(7)
(7)
When fluid enters the channel, it needs to travel some distance to attain its maximum velocity, Vmax, known as entry length and is given as (Abraham et al., Citation2020)
(8)
(8)
(9)
(9)
The pressure loss is a vital parameter, as it determines the power requirement of the pump to maintain the flow in the channel, and for the laminar flow in a circular channel, it is given as (Yunus, Citation2010)
(10)
(10)
3. Fabrication
This work uses the thin copper wire with a diameter of 240 µm as a mold for the channel. One can also select a wire of a different diameter to fabricate a channel according to their needs. The required components are as follows: Copper wire (240 µm diameter measured using a screw gauge), hot plate with temperature control, acetone, kitchen scrubs (steel wool preferred), aluminum foil, Sylgard 184 elastomer kit (PDMS), paper cup, desiccator, vacuum pump, spirit lamp or candle, ethanol, and a flexible rubber tube obtained from the Ramsons scalp vein set (size NG-20) by removing its needle.
Usually, copper wires come with a very thin coating of insulating material. These coatings may have an adverse effect on the sample or analyte used inside the channel. Hence, to remove the insulator coating from the copper wire, it is heated at 100 °C for 30 minutes, followed by cleaning using steel wool. Once the coating from the copper wire is removed, it is cleaned with acetone, followed by DI water, and dried on a hot plate at 100 °C for 5 minutes. After cleaning the copper wire, it is cut to a length of 8 cm for the straight channel. The mold for the channel is shown in . The wire length is 5.5 cm; as the wire is very thin, it is very difficult to keep it straight without any bending. One can use a wire of larger diameter to avoid any bending. The bending location of the wire are marked as point 1 to 6, and similar bending foci have been considered for simulation.
depicts the SEM and AFM representations of the mold used in fabrication of the channel. As seen in , the wire surface exhibits a defect-free quality. displays an image magnified at 200X, while offers a closer look at the wire surface at 1000X magnification. Furthermore, (c) reveals that the maximum surface roughness of the mold measures 134 nm, and this roughness is faithfully transferred to the channel wall during the soft lithography procedure. The influence of this 134 nm surface roughness varies depending on the application. For mixing applications, it can enhance fluid blending compared to channels with smooth walls, whereas in particle separation applications, it may lead to clogging.
Figure 2. Copper wire mold image (a) SEM image (Zoom 200X) (b) SEM image (Zoom 1000X) (c) AFM image (maximum surface roughness 134.4 nm).

It can be seen from that there are two small pieces of rubber tube, one at the inlet of the channel and the other at the outlet of the channel, having an inner diameter of 1500 µm ().
There is a huge mismatch in the diameter of the copper wire and the rubber tube. During fabrication, it is highly possible that PDMS will seep inside the rubber tube and block the inlet and outlet of the channel. To avoid PDMS getting inside the rubber tube, the end connecting to the channel mold is sealed with the help of hot tweezers. This rubber tube serves two purposes: first, it provides a perfect size for the inlet and outlet hole to interface the channel with the external world without using a biopsy punch, and second, it helps to pull out the wire from the cured PDMS without damaging it.
For minimum wastage of PDMS, a small plastic box is used as shown in . (a) shows the top view of the plastic box with a 4 × 7 array of holes on the cover made using a hot soldering iron rod. (b) shows the box in an open state. These holes on the box cover serve two purposes, as follows: first, they provide air passage during the degassing of the PDMS, and second, they help to keep the mold of the channels in proper position during the curing of PDMS so that they do not touch the bottom surface of the box.
Figure 4. Plastic container for optimal use of PDMS: (a) Top view, and (b) Box in the open state (Tiwari et al., Filed on 18th December Citation2023).

Once the box is ready, a thin layer of aluminum foil is placed in the plastic box as shown in ; it avoids the direct contact between the plastic and PDMS and hence, helps in easy removal of the cured PDMS from the plastic box without damaging it.
Figure 5. Plastic container with a layer of aluminum foil (Tiwari et al., Filed on 18th December Citation2023).

Without any support, the channel mold will touch the bottom surface of the box, and after curing of PDMS, the position of the mold will be very close to the surface, which may lead to a non-working channel after wire removal. To overcome this issue, the channel mold is attached to the holes made in the plastic box cover as shown in (a); this approach helps to keep the copper wire away from the bottom surface of the box. A mixture of PDMS base and bonder in the ratio of 10:1 is poured into the plastic container, and the cover is closed as shown in (b). One can also change the ratio of PDMS base and bonder to achieve stiffness of PDMS bulk, the ratio recommended by the manufacturer is 10 parts of PDMS base and 1 part of bonder.
Sometimes it may be possible that holes on the box cover are larger than the diameter of the rubber tube, and the mold does not fit in the hole properly. To overcome this issue, one may use small pieces of solder wire or toothpicks to attach the mold properly to the box cover. The box shown in (b) is placed inside the desiccator attached to a vacuum pump for degassing for 1 hour. Before taking out the box, it is confirmed that there are no trapped air bubbles in the PDMS mixture. In case there are air bubbles in the PDMS mixture, the container with the PDMS mixture should be kept in the vacuum until all air bubbles are removed. After completing the degassing process, the box is taken out from the desiccator and placed on a hot plate set to 90 °C for 1 hour for curing. After curing of PDMS, it is removed from the plastic container, all the aluminum foil is peeled off, and it is treated with ethanol. PDMS bulk bulges in ethanol, and hence, it is easy to remove the copper wire by mechanically pulling it out (Razavi Bazaz et al., Citation2020).
The final channel in the PDMS block is shown in . Wrinkles can be observed on the PDMS block, and this is due to the wrinkles present on the aluminum foil. Once the wire is removed safely from the PDMS bulk, tubing is performed with the help of superglue and Ramsons scalp vein set (size NG-20). While performing tubing, care must be taken to ensure that superglue does not enter the channel, or else it may contaminate the biological sample and block the channel. While performing the tubing, superglue got inside channel-1 and blocked it (). The best way of tubing is to first apply glue to the tube and hold the PDMS block in such a way that the inlet and outlet holes are facing towards the ground. After that, place the glued tube gently in the inlet and outlet holes and hold it for 1 minute; this technique results in working channels (channel-2 and channel-3). One thin copper wire can also be seen inside the PDMS block, which damaged the inlet of channel-4 while removing the copper wire.
4. Simulation
To understand the flow behaviour in the channel, FEM simulation is performed using COMSOL Multiphysics 6.0. Channel radius and length are set to 120 µm and 5.5 cm, respectively. In applications like continuous cell culture systems, a steady flow of fresh culture media is necessary. The length of time cells are exposed to these nutrients, which is determined by the residence time, is crucial for facilitating their growth and maintaining ideal conditions. Therefore, simulations are performed using two types of physics: laminar flow for steady-state simulations and particle tracing for time-dependent simulations. A physics-controlled fine mesh is used for the simulations, and a portion of the mesh model is shown in . Simulation involves the utilization of two types of channel models: a straight channel model () and a channel model incorporating bending ().
The inlet velocity is set to 24 × 10−3 m/s, and outlet pressure is set to zero. For the time-dependent simulation, start time, time step, and the end time are set to 0s, 0.05s, and 30s, respectively. On a computer with a Xeon(R) CPU E3, 4 cores, 16GB RAM, and the Windows 10 Enterprise operating system, it takes 5 minutes and 19 seconds, and 14 minutes and 55 seconds to complete the steady-state and time-dependent simulations for the straight channel model, and 3 minutes and 12 seconds, and 4 minutes and 8 seconds to complete the steady-state and time-dependent simulations for the channel with bending model, respectively.
5. Result and discussion
show the fluid velocity profiles as a function of the arc-length as predicted by the numerical simulation for straight and bent channel respectively. Velocity is constant at the inlet 0.0024 m/s, (distance 0 µm) for both the channel models, and as we progress along the length of the channel, there is a change in velocity (plots for distance 35 µm, 70 µm, and 105 µm show the developing flow). The arc length represents the channel diameter, and it can also be observed from that the plots at distances of 140 µm and 170 µm completely overlap on each other for both the models. Hence, it can be said that the entry length of the channel is 140 µm. The analytical value of the entry length, calculated using EquationEq. (9)(9)
(9) , is 144 µm, which is very close to the result obtained using simulation. The ratio of the entry length to channel diameter is 0.60. Alternatively, it can be said that the entry length is 0.60 times the diameter of the channel. Channel with bending () shows slightly higher velocity (0.004722 m/s) compared to straight channel (0.004693 m/s) and experiences higher friction loss than straight channel.
Figure 9. Velocity profile in the channel at various distance starting at 0 from inlet (a) Straight channel, and (b) Channel model with bending.

shows the velocity surface plot at a distance of 1 mm along the length of the channel from the inlet (straight channel), where the flow is fully developed. It has a good match with the velocity shown in . It can be observed from that the velocity at the channel wall is zero, and as we move from the channel surface towards the center of the channel, the velocity increases and obtains its maximum value at the center of the channel.
show the pressure drop along the length of the straight and bent channel respectively. It can be observed from that the maximum pressure required to maintain the average velocity of 0.0024 m/s in straight channel is 73.95 Pa. The analytical value of pressure drop obtained using EquationEq. (10)(10)
(10) is 73.45 Pa. Due to losses in channel due to bending (), the relative pressure drop is steeper.
To study the residence time of the fluid inside the microchannel, a time-dependent simulation is carried out with particles having their diameters and masses defined as 2.75 × 10−10 m and 2.989 × 10−26 kg, respectively, and the maximum number of particles is set to 10000. The transmission probability is obtained using COMSOL Multiphysics with Poincaré map as shown in .
Figure 12. Poincaré map at the outlet of the straight channel (a) Time 11.70s (b) Time 11.75s, and (c) Time 20.00s.

shows the outlet of the straight channel at the time 11.70s. It can be observed that none of the particles have reached the outlet of the channel, and the transmission probability is 0. From , it can be seen that only 0.3% of particles have reached the outlet of the channel at the time 11.75s. By substituting the maximum velocity in EquationEq. (7)(7)
(7) , the residence time is found to be 11.45s, which is very close to the result obtained from simulation. From , it is seen that the transmission probability is 0.832 at 20.00s, indicating that 83.2% of the particles have reached the outlet.
show the outlet of the channel with a bend at times 11.60s, 11.65s, and 20.00s, respectively. It can be observed that none of the particles have reached the outlet of the channel at 11.60s, resulting in a transmission probability of 0. In (b), it is evident that only 0.1% of the particles have reached the channel outlet by the time 11.70s. (c) displays a transmission probability of 0.629 at 20.00s, indicating that 62.9% of the particles have successfully reached the outlet. The variation in residence time between the channel with width bending and the straight channel is only 0.1s due to slightly higher velocity in the channel with bending.
Figure 13. Poincaré map at the outlet of the channel with bending (a) Time 11.65s (b) Time 11.70s, and (c) Time 20.00s.

To test the residence time of the channel, it is interfaced with a syringe pump (SKANRAY SP-205) and a 5 ml DISPOVAN syringe is used to supply the dye. The flow rate for the DISPOVAN 5 ml syringe is set to 0.36 ml/hr, equivalent to the volumetric flow rate 1.0257 × 10−10 m3/s. It can be seen from (a) that fluid enters the channel at 1 minute 27.55 seconds (labeled as A), it fills half of the channel around 1 minute 31.29 seconds shown in (labeled as B), and the fluid comes out from the channel at 1 minute 40.29 seconds, shown in (labeled as ). The difference in time shown in and gives the residence time of 12.71 seconds for the channel. This is higher than the result obtained from the simulation. This difference is due to the variation of the wire diameter from 240 µm to 247 µm. Channel-2 is used for SEM imaging, and hence, no residence time testing is performed with it.
Figure 14. Residence time testing of channel (a) Empty channel (b) Half-filled channel, and (c) Completely filled.

shows the SEM image of the channel cross-section. It can be seen from that the channel diameter is around 244.3 µm.
lists the microchannels fabricated in PDMS material having rectangular/circular cross-sections reported by different researchers and their applications. We believe that the channel reported in this article may have potential applications in the various studies of biomedical analysis.
Table 3. Microfluidic channel and their applications.
6. Conclusion
The design and fabrication of a microfluidic channel with a circular cross-section using a ‘do-it-yourself’ approach has been successfully demonstrated, which is both safe and suitable for small research groups with minimal infrastructure. The soft-lithography method was used to fabricate the channel in PDMS using a copper wire mold with a diameter of 240 µm, and the channel was created with the wire pull-out method. The channel diameter was confirmed to be the same as the mold diameter with the help of SEM imaging. This work addresses the optimal use of PDMS for channel fabrication, as well as the challenges faced when connecting the microchannel to the external world via tubing and techniques to overcome them. Through analytical, simulation, and test results, we demonstrated a good match between our findings.
Acknowledgements
The authors of this paper would like to thank Dr. Shounak De, an Additional Professor at the same department, for providing access to his laboratory equipment. The authors would also like to acknowledge I-STEM, Indian Institute of Science, CeNSE, Bengaluru, Karnataka 560012, for providing access to COMSOL Multiphysics 6.0 for simulating the design. Lastly, the authors are thankful to Dr. Swapna K S, an Assistant Professor (Senior Scale) at the Department of Humanities and Management, MIT, MAHE, Manipal, for her contribution towards language correction.
Disclosure statement
No potential conflict of interest was reported by the author(s).
Additional information
Notes on contributors
Shailendra Kumar Tiwari
Shailendra Kumar Tiwari earned his Ph.D. in Microfluidics and MEMS for biomedical applications from Manipal Academy of Higher Education, Karnataka. He holds a M.Tech in VLSI Design from Sathyabama University, Tamil Nadu. Currently, an Associate Professor at Manipal Institute of Technology, his research focuses on MEMS and VLSI Design.
Somashekara Bhat
Somashekara Bhat is a Professor in the Department of Electronics and Communication Engineering at Manipal Institute of Technology, MAHE, Karnataka. He earned his Ph.D. in MEMS from the Indian Institute of Technology, Madras, in 2008. His research focuses on MEMS and electronics for biomedical applications.
Sandhya Parasnath Dubey
Sandhya Parasnath Dubey holds a Ph.D. in bioinformatics and high-performance computing from Manipal Academy of Higher Education, Karnataka. With an M.E. in CSE from Sathyabama University, Tamil Nadu, she is currently an Assistant Professor in the Department of Data Science and Computer Applications at Manipal Institute of Technology, MAHE, Manipal, India.
References
- Abdelgawad, M., Wu, C., Chien, W. Y., Geddie, W. R., Jewett, M. A., & Sun, Y. (2011). A fast and simple method to fabricate circular microchannels in polydimethylsiloxane (PDMS). Lab on a Chip, 11(3), 545–551. https://doi.org/10.1039/C0LC00093K
- Abraham, J. P., Gorman, J. M., & Minkowycz, W. J. (2020). Advances in heat transfer. Academic Press.
- Ali, M., Srivastava, S., Solanki, P. R., Reddy, V., Agrawal, V. V., Kim, C., John, R., & Malhotra, B. D. (2013). Highly efficient bienzyme functionalized nanocomposite-based microfluidics biosensor platform for biomedical application. Scientific Reports, 3(1), 2661. https://doi.org/10.1038/srep02661
- Amirifar, L., Besanjideh, M., Nasiri, R., Shamloo, A., Nasrollahi, F., de Barros, N. R., Davoodi, E., Erdem, A., Mahmoodi, M., Hosseini, V., Montazerian, H., Jahangiry, J., Darabi, M. A., Haghniaz, R., Dokmeci, M. R., Annabi, N., Ahadian, S., & Khademhosseini, A. (2022). Droplet-based microfluidics in biomedical applications. Biofabrication, 14(2), 022001. https://doi.org/10.1088/1758-5090/ac39a9
- Choi, J. S., Piao, Y., & Seo, T. S. (2013). Fabrication of a circular PDMS microchannel for constructing a three-dimensional endothelial cell layer. Bioprocess and Biosystems Engineering, 36(12), 1871–1878. https://doi.org/10.1007/s00449-013-0961-z
- Effati, E., & Pourabbas, B. (2018). New portable microchannel molding system based on micro-wire molding, droplet formation studies in circular cross-section microchannel. Materials Today Communications, 16, 119–123. https://doi.org/10.1016/j.mtcomm.2018.05.006
- Eftekhari, A., Alipour, M., Chodari, L., Maleki Dizaj, S., Ardalan, M., Samiei, M., Sharifi, S., Zununi Vahed, S., Huseynova, I., Khalilov, R., Ahmadian, E., & Cucchiarini, M. (2021). A comprehensive review of detection methods for SARS-CoV-2. Microorganisms, 9(2), 232. https://doi.org/10.3390/microorganisms9020232
- Gautam, G. P., Burger, T., Wilcox, A., Cumbo, M. J., Graves, S. W., & Piyasena, M. E. (2018). Simple and inexpensive micromachined aluminum microfluidic devices for acoustic focusing of particles and cells. Analytical and Bioanalytical Chemistry, 410(14), 3385–3394. https://doi.org/10.1007/s00216-018-1034-6
- Hassanpour-Tamrin, S., Sanati-Nezhad, A., & Sen, A. (2021). A simple and low-cost approach for irreversible bonding of polymethylmethacrylate and polydimethylsiloxane at room temperature for high-pressure hybrid microfluidics. Scientific Reports, 11(1), 4821. https://doi.org/10.1038/s41598-021-83011-8
- Kandlikar, S., Garimella, S., Li, D., Colin, S., & King, M. R. (2005). Heat transfer and fluid flow in minichannels and microchannels. Elsevier.
- Kim, S., Kim, J., Joung, Y. H., Choi, J., & Koo, C. (2018). Bonding strength of a glass microfluidic device fabricated by femtosecond laser micromachining and direct welding. Micromachines, 9(12), 639. https://doi.org/10.3390/mi9120639
- Li, G., & Xu, S. (2015). Small diameter microchannel of PDMS and complex three-dimensional microchannel network. Materials & Design, 81, 82–86. https://doi.org/10.1016/j.matdes.2015.05.012
- Liu, C. (2012). Foundations of MEMS. Pearson Education India.
- Oliveira, N. M., Vilabril, S., Oliveira, M. B., Reis, R. L., & Mano, J. F. (2019). Recent advances on open fluidic systems for biomedical applications: A review. Materials Science & Engineering, 97, 851–863. https://doi.org/10.1016/j.msec.2018.12.040
- Paiè, P., Zandrini, T., Vázquez, R. M., Osellame, R., & Bragheri, F. (2018). Particle manipulation by optical forces in microfluidic devices. Micromachines, 9(5), 200. https://doi.org/10.3390/mi9050200
- Pinto, E., Faustino, V., Rodrigues, R. O., Pinho, D., Garcia, V., Miranda, J. M., & Lima, R. (2014). A rapid and low-cost nonlithographic method to fabricate biomedical microdevices for blood flow analysis. Micromachines, 6(1), 121–135. https://doi.org/10.3390/mi6010121
- Preetam, S., Nahak, B. K., Patra, S., Toncu, D. C., Park, S., Syväjärvi, M., Orive, G., & Tiwari, A. (2022). Emergence of microfluidics for next generation biomedical devices. Biosensors and Bioelectronics, 10, 100106. https://doi.org/10.1016/j.biosx.2022.100106
- Qi, W., Zheng, L., Wang, S., Huang, F., Liu, Y., Jiang, H., & Lin, J. (2021). A microfluidic biosensor for rapid and automatic detection of Salmonella using metal-organic framework and Raspberry Pi. Biosensors & Bioelectronics, 178, 113020. https://doi.org/10.1016/j.bios.2021.113020
- Rathod, M. L., Ahn, J., Jeon, N. L., & Lee, J. (2017). Hybrid polymer microfluidic platform to mimic varying vascular compliance and topology. Lab on a Chip, 17(14), 2508–2516. https://doi.org/10.1039/C7LC00340D
- Razavi Bazaz, S., Rouhi, O., Raoufi, M. A., Ejeian, F., Asadnia, M., Jin, D., & Ebrahimi Warkiani, M. (2020). 3D printing of inertial microfluidic devices. Scientific Reports, 10(1), 5929. https://doi.org/10.1038/s41598-020-62569-9
- Sackmann, E. K., Fulton, A. L., & Beebe, D. J. (2014). The present and future role of microfluidics in biomedical research. Nature, 507(7491), 181–189. https://doi.org/10.1038/nature13118
- Shepherd, S. J., Issadore, D., & Mitchell, M. J. (2021). Microfluidic formulation of nanoparticles for biomedical applications. Biomaterials, 274, 120826. https://doi.org/10.1016/j.jbiomech.2015.11.031
- Song, S. H., Lee, C. K., Kim, T. J., Shin, I. C., Jun, S. C., & Jung, H. I. (2010). A rapid and simple fabrication method for 3-dimensional circular microfluidic channel using metal wire removal process. Microfluidics and Nanofluidics, 9(2–3), 533–540. https://doi.org/10.1007/s10404-010-0570-y
- Tiwari, Shailendra Kumar, Dubey, Sandhya Parasnath, Bhat, Somashekara, Microfluidic Channel Integrated With Wire Microheater and Temperature Sensor and Method for Its Fabrication Thereof, Indian Patent Office, Chennai. Application No. 202341086514., Filed on 18th December 2023
- Tiwari, S. K., Bhat, S., & Mahato, K. K. (2020). Design and fabrication of low-cost microfluidic channel for biomedical application. Scientific Reports, 10(1), 9215. https://doi.org/10.1038/s41598-020-65995-x
- Wang, X., Sun, Q., & Pei, J. (2018). Microfluidic-based 3D engineered microvascular networks and their applications in vascularized microtumor models. Micromachines, 9(10), 493. https://doi.org/10.3390/mi9100493
- Yin, B., Wan, X., Sohan, A. M. F., & Lin, X. (2022). Microfluidics-based POCT for SARS-CoV-2 diagnostics. Micromachines, 13(8), 1238. https://doi.org/10.3390/mi13081238
- Yunus, A. C. (2010). Fluid Mechanics: Fundamentals and Applications (Si Units). Tata McGraw Hill Education Private Limited.