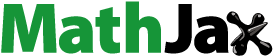
Abstract
The design and performance evaluation of a 30 kN mechanical testing machine with capacities for room temperature and hot tensile and compression testing was performed. The design philosophy, material selection, and construction strategy were based on the functions and properties of the materials and control systems, local availability, cost considerations, and ease of assembly and decoupling of the parts. Simulation studies were conducted to optimize the design, loading capacity, and specimen dimensions and configurations. The loading mechanism was by a hydraulic system and a loading frame was constructed to house the hydraulic loading, specimen holding, and heating band systems. Testing to ascertain the performance of the machine using aluminum 6063 alloy samples was preceded by load cell and extensometer calibration of the machine. From the performance evaluation, the UTS and percent elongation values for room temperature (25 °C) tensile test (446–454 MPa and 28.3–28.7%) hot tensile tests at 250 °C (232–233 MPa and 36.3–36.7%), room temperature compression test (439–518 MPa and 32.7–33%), and hot compression test at 250 °C (226–233 MPa and 34–35%), were found to be largely reproducible and values were within the range reported in literature for Aluminum 6063 alloy. The softening mechanisms dominant at elevated temperatures ensured that the strain hardening effect was minimal, which is the reason for the superior percent elongation but lower UTS values for tensile and compression tests performed at 250 °C.
1. Introduction
The adoption of material testing as a means of product validation and quality assurance is very instructive in engineering component and part development. For materials intended for structural and load bearing application, mechanical testing which enables the determination of mechanical strength, toughness, ductility, and other deformability parameters, are crucial to safeguard against premature and costly failures in service (Komvopoulous, Citation2017; Sehhat et al., Citation2022). Quality control and research and development units of many manufacturing companies can hardly certify structural products for commercialization without records of their mechanical properties (Astakhov, Citation2018; Baharuddin and Yani, 2018). Hence there is a growing need for mechanical testing facilities, especially with specifications that permit ease of operation, precision, and accuracy of results. Consequently, there have been several studies carried out to develop mechanical testing facilities that meet the aforementioned criteria. Tiernan and O’Connor (Tiernan and O’Connor, Citation2018) developed a mechanical testing device with the capacity to conduct tensile and compression testing of metallic materials at elevated temperatures. The device, which consists of an environmental chamber, specimen gripping mechanism, and accompanying instrumentation, can perform tensile testing on notched and unnotched samples, and compression tests at temperatures up to 1200 °C. Stoudt et al. (Citation2017) developed an in-plane measurement technique that can be utilized to provide performance data required to validate complex predictions of work hardening behavior during reversed uniaxial deformation of thin sheet metal specimens subjected to bidirectional loading. Merklein et al. (Citation2016) developed an in-plane shear testing machine that compares the deformation response of the test material to forces applied tangentially. The machine tests are applied primarily to thin sheet materials, either metals or composites, such as fiber-reinforced polymers. Several other mechanical testing machines that permit tension-compression testing in metallic materials, and multiaxial testing of materials with varied configurations, among others, have been reported in the literature (Bhaskar et al., Citation2021; Medellín and De la Peña, Citation2017; Thoerner et al., Citation2018). However, most of these machines are developed outside of sub-Saharan Africa.
In recently times, most sub-Saharan African countries are striving to improve on their production capability especially in manufactured engineering products required for structural applications. There is however, paucity of know-how in the area of development of mechanical testing facilities for product testing. Most of the companies and research and development institutions rely on imported facilities to perform various test desired for product development and quality control (Alaneme and Asemewanlen, Citation2021). However, a good number of these mechanical testing facilities are purchased at highly exorbitant cost due to high exchange rate between local currencies and international transactional currencies like the dollar, pound Sterling or Euro. In addition, the instrumentation sensitivity, operational protocol, and power requirements of many imported mechanical testing facilities are not completely adjustable and supported by the infrastructure in our environment for effective operation, leading to short service life. When this occurs, getting the appropriate replacement parts, official procurement policies, cost implications and delivery dates can make these facilities moribund for long periods.
There is also the unfortunate dimension to the problem where the facilities are supplied with incomplete or incompatible parts which make operation at point of delivery impracticable. It is thus imperative and of necessity for local solutions to address this crucial need in the engineering and manufacturing sector of most African countries to be given attention. To address the issue, there have been some attempts to develop mechanical testing facilities locally.
The present work is aimed at the development of mechanical testing machine with multifunctional capability for room and elevated temperature (up to 400 °C) tensile and compression testing of materials. Several local efforts have been put in place to develop mechanical testing facilities in Nigeria but none to the best of our knowledge has been developed with capacity and operational mechanism to facilitate both room temperature and elevated temperature testing at quasi-static and variable loading rates. It is expected that the design of this machine will help address the acute need for reliable, cost-effective, and easy to maintain mechanical testing facility for our universities, research institutes and centers, and construction and manufacturing establishments
2. Materials and method
2.1. Materials selection
The materials used for the development of the machine, include: mild steel sheets, steel rods, alloy steel profiles of assorted sizes, electric motors of low revolution per minute (rpm), gear reducer, 50 kN rated Load cell, self-alignment nuts, sensors, pipes, bearings, gripping chucks, counters, heater band, crank and connecting rod assembly. The main parts of this design are the machine frame, traveling shafts, threaded shaft, lower chuck assembly, sample holder and hydraulic system. The upper and lower chuck assembly, and sample holder system, are produced from medium carbon low alloy steel, while the traveling shaft, threaded shaft and vibration shaft are of nickel carbon steel.
2.2. Design simulation
The simulation design of the machine showing the entire outlook of the machine is presented in , while the essential parts of the machine that include the base casing, plain steel rod, sample holders, electric motor, top and base casings, threaded rod, and sample griper, are presented in . The deformation load of 30 kN was used to determine the shaft, specimen, and sample holder dimensions, which were used to simulate the working of the machine in tension and compression ( and ). The simulation analysis was done using solidworks software, which is embedded with structural analysis tools that utilize finite element analysis to predict materials physical behavior. In the simulation analysis conducted, the design optimization performed made it possible to assure that the load cell capacity is adequate for the tensile and compression loading to fracture of the samples, without deformation of the sample holder and loading frames when in operation.
2.3. Design
The following calculations were factored in the design and materials/components.
2.3.1. Design of shaft
Due to its machinability and strength, four solid mild steel shaft of diameter 30 mm was used to support the sample holder (Amiebenomo and Yesufu, Citation2013) using EquationEq. (1)(1)
(1) .
(1)
(1)
where
F, and A are stress (MPa), Force (N) and Area (mm2), respectively.
Polar moment of inertia of the shaft was also calculated using EquationEq. (2)(2)
(2) .
(2)
(2)
where J is the polar moment of inertia and d is the shaft diameter.
2.3.2. Specimen dimension and machine capacity
EquationEquation (3)(3)
(3) was used to calculate the specimen diameter.
(3)
(3)
where T is the torque, d is the specimen diameter, and
is the shear yield stress; and assuming Tresca’s yield criterion, the shear yield stress is given as 350 MPa while constant divisible factor is 16 (Gbasouzor et al., Citation2013).
Therefore, the maximum operating machine capacity for this machine is 40 kN.
2.4. Construction and assembly
The frame of the multifunctional testing machine was constructed with the following units: the base design for the hydraulic housing, the hydraulic system assembly, the supporting shaft framing assembly, the machine supports and the control panel assembly. Construction of the hydraulic stand with frame were carried out along with the specimen holder fabrication. The assembly was made firm using double bolting system. Machining of the shafts were carried for bearings seating. The heater band assembly was also constructed to 400 °C maximum temperature.
The two chrome-base steel rods were connected with toughened 22-in. bolts to one of the 40 mm thick plate and was assembled on the hydraulic mobile head without welding. Holes were created on the thick plate to allow the threaded shafts free longitudinal movement during operation of the machine. Bearings were perfectly positioned on the top of the shafts while the heater band handle was slide into one of the chrome steel shafts before fixing the housing top cover into place. The machine after construction, assembly and painting is presented in .
2.5. The block diagram of the mechanical testing machine
The block diagram for the electrical units of the machine is presented in . It comprises of the load tension or stress unit made of load cell, load cell amplifier with 24 bits serial out analog- to digital-converter (ADC), extensometer which comprises of 100 mm long linear potentiometer and 16 bits ADC (ADS 1115) and motor movement control unit. The secure digital (SD) card for data storage, LCD for display information of activities taken place in the system and micro-controller to co-ordinate all the activities together.
2.6. Machine calibration
The standardization of load cell unit was done by placing a standard load on the load cell in increasing order and taking record of the measured load value displayed on the machine to assure accuracy of the readings from the machine. In addition, extensometer calibration was performed before testing was carried out. The extensometer is a linear potentiometer () with two ends of a straight resistor connected across the source voltage. A sliding contact can be slide on the resistor through a track attached along the resistor. The terminal connected to the sliding is connected one end of the output circuit and one of the terminals of the resistor is connected to the other end of the output circuit. To determine distance of elongation (strain) of sample material via extensometer (Ewetumo, Citation2019).
(4)
(4)
(5)
(5)
where q in millimeter per volt (mm/V) is proportionality constant.
Substituting Eq. (14) in Eq. (15).
(6)
(6)
ADS1115 full scale and the value of bit are determined by the Programmable Gain Amplifier (PGA) setting. This differs from the Arduino where full scale is determined by a reference voltage in the default mode, the setting is +/−6.144 V. For better extensometer reading another PGA setting, can be obtained as full scale of +/−2.048 V. That provides a resolution of 0.0635 mV. The Arduino microcontroller uses an ADS library to the operation of ADS1115. To wire the ADS1115 to the microcontroller via SDA and SCL protocol. The output of the pot is link to A0 of ADS1115. The Vref used is 2.048 V (Ewetumo, Citation2019; Osinowo et al., Citation2020).
2.7. Testing and basis for performance evaluation
2.7.1. Tensile testing
The testing of the machine was done at room temperature and at 250 °C using Al 6063 samples machined to dimensions 6 mm gauge diameter and gauge length of 40 mm having grip length and diameter of 10 mm. The room temperature tests were performed at a quasi-static strain rate of 10−3/s and the samples were pulled to fracture. Six repeat tests were conducted on the samples in order to establish the reproducibility and repeatability of results. The testing procedure and data analysis were in accordance with ASTM 8 M (2022) standard. Similarly, hot tensile testing conducted at 250 °C was performed on Al 6063 samples also machined with configuration as described for the room temperature test. The samples were mounted on the sample holder and the heater band was set in place. The samples were heated and allowed to stabilize at the test temperature before the sample was pulled to fracture at a strain rate of 10−3/s. The testing and data analysis procedures were in accordance with ASTM E21 (Citation2020) standard. Six repeat tests were also performed on the samples for test reliability to be established.
2.7.2. Compression testing
The Al 6063 samples were machined to gauge length of 15 and 10 mm diameter for the room and elevated temperatures compression testing. For the room temperature test, the samples were mounted and held in place by the sample holder and the extensometer was connected to take readings as compression of the sample was performed. The compression and displacement readings were taken and the data analyzed in accordance with ASTM E9 (ASTM E9-09, XXXX) standard. In addition, hot compression testing was conducted at 250 °C on Al 6063 samples which were machined with configuration as described for the room temperature compression test. The samples were mounted on the sample holder and the heater band was set in place. The samples were heated and allowed to stabilize at the test temperature before the sample was compressed at a strain rate of 10−3/s. The hot compression testing procedure and data analysis method were in accordance with ASTM E209 (ASTM E209-18, XXXX) standard. Six repeat tests were conducted following the same testing procedures to establish testing reliability and accuracy of results. During the elevated temperature testing, care was exercised to ensure that the sample does not touch the heater band.
3. Results and discussion
3.1. Tensile testing
The results of the tensile tests conducted at room and elevated temperatures are presented in and . From , it is observed that the samples exhibited similar flow characteristics and plastic flow behavior. The samples exhibited progressive strain hardening until the UTS is attained as reflected in the ultimate tensile strength and % elongation values which ranged between 446–454 MPa and 28.3–28.7%, respectively. This is an indication that the values from repeat tests are consistent and reliable hence the calibration of the machine is in order. It is also noted that the values obtained are consistent with values of UTS and % elongation for Al 6063 reported in literature (Alaneme and Aluko, Citation2012). From , it is observed that the UTS and % elongation values ranged between 232 and 233 MPa and 36.3–36.7%, respectively, which are within the range for similarly hot tensile deformed Al alloys reported in literature (Dai et al., Citation2020). The values from the repeat tests show that the machine readings are reproducible and accurate. On comparing the room temperature UTS values with that at 250 °C, it is noted that the room temperature values are higher than that at 250 °C. This is expected as more dislocation pile up and multiplication occurs at room temperature than at 250 °C where dislocation annihilation by dynamic recovery is more dominant (Alaneme and Okotete, Citation2019). The deformation mechanisms dominant at elevated temperatures ensure that strain hardening effect is minimal, which is why little difference in the UTS and fracture stress is observed in . In contrast, the % elongation values of the room temperature tested samples are lower than that of the samples tested at 250 °C. This is due to the lower capacity of dislocations to circumvent barriers posed by boundaries and other dislocations generated during the deformation process. The lower rate of dislocation redistribution and annihilation that occurs at room temperature relative to higher temperatures, results in the presence of more obstructions to dislocation movement, which translates to reduced flow capacity (ductility) at room temperature (Courtney, Citation2006; Wu et al., Citation2021).
3.2. Compression testing
The results of the compression tests conducted at room and elevated temperatures are presented in and . From , it is observed that the samples exhibited similar flow characteristics and plastic flow behavior. The samples exhibited progressive strain hardening until the peak stress is attained, albeit the values varied slightly for the repeat tests performed. The ultimate compressive strength and % elongation values ranged between 439 and 518 MPa and 32.7 and 33%, respectively. This is an indication that the values from repeat tests showed some degree of scatter for the compression strength; however, the % elongation were within the same range for all the repeat test samples. The values of the compressive strength were generally within the range reported in literature for Al 6063 (Saravanakumar et al., Citation2014). From , it is observed that the compressive strength and % elongation values ranged between 226–233 MPa and 34–35%, respectively for the hot compressed samples. These values fall within the range reported in literature for Al based systems hot compressed at 250 °C (Alaneme et al., Citation2021). The values from the repeat tests show that the machine readings are largely reproducible and accurate at elevated temperature than at room temperature. On comparing the room temperature compressive strength values with that at 250 °C, it is noted that the room temperature values are higher than that at 250 °C. This is expected as more dislocation pile up and multiplication occurs at room temperature than at 250 °C where partial annihilation of dislocations by dynamic recovery is more dominant (Bembalge and Panigrahi, Citation2021). The deformation mechanisms dominant at elevated temperatures ensure that strain hardening effect is minimal, which is why little difference in the compressive strength and fracture stress is observed. In addition, the % elongation values of the room temperature tested samples are slightly lower than that of the samples tested at 250 °C. This is due to the lower capacity of dislocations to circumvent barriers posed by the boundaries and other dislocations generated during the deformation process. The lower rate of dislocation redistribution and annihilation that occurs at room temperature relative to higher temperatures, results in the presence of more obstructions to dislocation movement, which translates to reduced flow capacity (ductility) at room temperature.
4. Conclusion
The development and performance evaluation of a 30 kN mechanical testing machine with capacities for room temperature and hot deformation tensile and compression testing, was undertaken. From the research, the following conclusions are drawn:
Simulation studies aided the optimization of the machine design for load capacity, sample holder and specimen dimensions and configurations.
The UTS and percent elongation values for room temperature (25 °C) tensile test (446–454 MPa and 28.3–28.7%) hot tensile tests at 250 °C (232–233 MPa and 36.3–36.7%), room temperature compression test (439–518 MPa and 32.7–33%), and hot compression test at 250 °C (226–233 MPa and 34–35%), were found to be largely reproducible and values were within the range reported in literature for Aluminum 6063 alloy.
The deformation mechanisms dominant at elevated temperatures ensured that strain hardening effect is minimal, which is the reason of the superior percent elongation but lower UTS values for tensile and compression tests performed at 250 °C.
Acknowledgement
The authors acknowledge the grant support received from the Nigerian Tertiary Education Trust Fund (TETFund) Institutional Based Research (IBR), which enabled the completion of this research. The authors equally appreciate the support of their institutions and the National Research Foundation of South Africa.
Disclosure statement
No potential conflict of interest was reported by the authors.
Data availability statement
The data used for this study will be provided on request.
References
- Alaneme, K. K., & Aluko, A. O. (2012). Fracture toughness (K1C) and tensile properties of as-cast and age-hardened aluminium (6063)–silicon carbide particulate composites. Scientia Iranica, 19(4), 992–996. https://doi.org/10.1016/j.scient.2012.06.001
- Alaneme, K. K., & Asemewanlen, E. K. (2021). J-evaluation on tensile testing (JETT) based fractured toughness assessment of aluminum (6063) alloy using circumferentially round bar specimens. Materials Today: Proceedings, 38, 724–728. https://doi.org/10.1016/j.matpr.2020.04.060
- Alaneme, K. K., & Okotete, E. A. (2019). Recrystallization mechanisms and microstructure development in emerging metallic materials—a review. Journal of Science: Advanced Materials and Devices, 4(1), 19–33. https://doi.org/10.1016/j.jsamd.2018.12.007
- Alaneme, K. K., Babalola, S. A., Chown, L. H., Maledi, N. B., & Bodunrin, M. O. (2021). Study on hot deformation behavior and workability of stir-cast Al6063-6wt.% steel p-based composites. Journal of the Mechanical Behavior of Materials, 30(1), 110–117. https://doi.org/10.1515/jmbm-2021-0012
- Amiebenomo, S. O., & Yesufu, S. (2013). The design and fabrication of compression and extension testing machine. Asian Review of Mechanical Engineering, 2(1), 28–34. https://doi.org/10.51983/arme-2013.2.1.2326
- Astakhov, V. P. (2018). Mechanical Properties of Engineering Materials: Relevance in Design and Manufacturing. In: J. Davim (Ed.), Introduction to mechanical engineering. Materials forming, machining and tribology. Springer.
- ASTM E209-18. Standard Practice for Compression Tests of Metallic Materials at Elevated Temperatures with Conventional or Rapid Heating Rates and Strain Rates. ASTM International.
- ASTM E21 (2020). Standard Test Methods for Elevated Temperature Tension Tests of Metallic Materials. ASTM International.
- ASTM E8M Standard. (2022). Standard Test Methods for Tension Testing of Metallic Materials., ASTM International.
- ASTM E9-09. Standard Test Methods of Compression Testing of Metallic Materials at Room Temperature. ASTM International.
- Baharuddin, B., & Yani, I. (2018). Implementation of design and development of tensile testing machine for application in soft material. Journal of Multidisciplinary Engineering Science and Technology (JMEST), 5(12), 9263–9267.
- Bembalge, O. B., & Panigrahi, S. K. (2021). Hot deformation behavior and processing map development of cryorolled AA6063 alloy under compression and tension. International Journal of Mechanical Sciences, 191, 106100. https://doi.org/10.1016/j.ijmecsci.2020.106100
- Bhaskar, L. K., Kumar, G., Srinivasan, N., & Kumar, R. (2021). Design and development of a miniaturized multiaxial test setup for in situ x-ray diffraction experiments. Review of Scientific Instruments, 92(1), 015116. https://doi.org/10.1063/5.0031805
- Courtney, T. H. (2006). Mechanical Behaviour of Materials (2nd ed.). Overseas Press.
- Dai, P., Luo, X., Yang, Y., Kou, Z., Huang, B., Zang, J., & Ru, J. (2020). High temperature tensile properties, fracture behaviors and nanoscale precipitate variation of an Al–Zn–Mg–Cu alloy. Progress in Natural Science: Materials International, 30(1), 63–73. https://doi.org/10.1016/j.pnsc.2020.01.007
- Ewetumo, T. (2019). Development of a low-cost penetrometer with depth measurement. International Journal Scientific Research and Development, 7(4), 483–486.
- Gbasouzor, A. I., Okeke, O. C., & Onyebuchi, C. L. (2013). Design and characterization of a fatigue testing machine. Proceedings of the World Congress on Engineering and Computer Science, 1, 97–115.
- Komvopoulous, K. (2017). Mechanical testing of engineering materials (2nd ed.). Cognella Academic Publishing.
- Medellín, L. F. P., & De la Peña, J. Á. D. (2017). Design of a biaxial test module for Uniaxial Testing Machine. Materials Today: Proceedings, 4(8), 7911–7920. https://doi.org/10.1016/j.matpr.2017.07.127
- Merklein, M., Lechner, M., Gröbel, D., Löffler, M., Schneider, T., Schulte, R., & Hildenbrand, P. (2016). Innovative approaches for controlling the material flow in sheet-bulk metal forming processes. Manufacturing Review, 3(2), 2. https://doi.org/10.1051/mfreview/2016001
- Osinowo, M. O., Willoughby, A. A., Dairo, O. F., Ewetumo, T., & Kolawole, L. B. (2020). Development of ultra low-cost data acquisition system (DAQ) for developing countries. International Journal of Engineering Research & Technology, India, 9(09), 135–142.
- Saravanakumar, A., Sasikumar, P., & Sivasankaran, S. (2014). Synthesis and mechanical behavior of AA 6063-x wt. % Al2O3-1% Gr (x = 3, 6, 9 and 12 wt.%) hybrid composites. Procedia Engineering, 97, 951–960. https://doi.org/10.1016/j.proeng.2014.12.371
- Sehhat, M., Mahdianikhotbesara, A., & Hadad, M. (2022). Formability investigation for perforated steel sheets. SAE International Journal of Materials and Manufacturing, 15(2), 175–186. https://doi.org/10.4271/05-15-02-0012
- Stoudt, M. R., Levine, L. E., & Ma, L. (2017). Designing a uniaxial tension/compression test for springback analysis in high-strength steel sheets. Experimental Mechanics, 57(1), 155–163. https://doi.org/10.1007/s11340-016-0202-x
- Thoerner, R. P., King, J. D., & Saunders, M. M. (2018). Application of design aspects in uniaxial loading machine development. Journal of Visualized Experiments, 139, 58168. https://doi.org/10.3791/58168
- Tiernan, P., & O’Connor, G. (2018). Design, manufacture and test of a high temperature tensile and compression testing device. Procedia Manufacturing, 17, 672–679. 28th International Conference on Flexible Automation and Intelligent Manufacturing (FAIM2018), June 11–14, 2018, Columbus, OH, USA. https://doi.org/10.1016/j.promfg.2018.10.116
- Wu, X., Jiang, P., Chen, L., Yuan, F., & Zhu, Y. (2021). Extraordinary Strain Hardening by Gradient Structure, Heterostructured Materials (1st ed., pp. 19). Imprint Jenny Stanford Publishing.