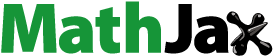
Abstract
Carbon fabric-reinforced polymer (CFRP) composites have replaced most aluminum and ferrous alloys in aerospace construction owing to their attractive high strength-to-weight ratio. CFRPs used in aircraft should have electromagnetic shielding ability in addition to structural properties. Hence, the study of Electromagnetic (EM) wave shielding by hybrid CFRPs is an important topic of research. In this article, we summarize research advancements for enhancing the EM wave shielding of CFRP composites in the X-band frequency range. This study focuses on the manufacturing and performance of carbon-fabric-based composites with metals, metal oxides, and carbon-based fillers. The factors influencing the EM wave shielding ability of the CFRP composites are also discussed. The EM wave shielding of Carbon fibers (CF) was remarkably improved by coating, electroless plating, and synthesizing nanoparticles on the carbon fiber surface. It was found that the carbonious fillers and metal nanofillers such as Nickel (Ni), Iron (Fe), and Silver (Ag)-loaded multilayer CFRP composites showed better absorption modes of EM wave shielding with good structural properties. Owing to the Ag nanowire coating on the CFs, the shielding effectiveness was drastically improved. However, in addition to the type of filler, the structural properties are also influenced by the manufacturing method and type of composite. In the case of laminated composites, the absorption-laminated EM shielding is influenced by the architecture of the plys and the type and number of interplay in the composite construction. In addition, the techniques adopted for the synthesis of nanoparticles and dispersion in composites for effective EM shielding are challenging tasks.
Reviewing Editor:
1. Introduction
EM waves consist of electric and magnetic fields vibrating perpendicular to each other and traveling at the speed of light. EM waves include radio, microwaves, infrared, visible, ultraviolet, X-rays, and gamma rays. EM waves are emitted from both artificial and natural sources. The electromagnetic wave spectrum and emission sources are shown in .
Figure 1. The spectrum of electromagnetic radiation, corresponding sources, and devices (Jagadeesh Chandra et al., Citation2019).

Electronic gadgets, communication antennas, and control systems used in military applications, industry, and domestic purposes are prominent artificial electromagnetic (EM) radiation sources. In addition, natural sources such as lightning, thunderstorms, and electrostatic discharge are also sources of EM radiation. Of the above two types of sources, the interactions of artificial sources with human beings and the environment have increased due to the application of advanced technology in daily life.
EM radiation harms humans, birds, and other animals on the Earth. The interaction of EM radiation emitted from the above sources with the dielectric biological tissues of plants, animals, and humans causes a magnetic effect. For example, suppose that a phone conversation continues for a longer time. In this case, the body tissues are heated by the EM radiation emitted by the phone, and the fluid molecules in the body tissues are heated. This can cause tissue damage and tumors. In addition, high-frequency EM radiation deeply penetrates animal and human skin. It also damages the internal tissues and organs (Stein et al., Citation2015). Exposure of humans and animals to prolonged and continuous non-ionizing radiation leads to DNA errors (Diem et al., Citation2005). Balodis et al. (Citation1996) showed that tree growth diminishes due to continuous exposure to EM radiation. Monselise et al. (Citation2011) demonstrated that alanine accumulation in plants was reduced by exposure of plants to EM radiation. In addition, the EM waves emitted from one electronic device interfere with the neighboring devices, leading to electromagnetic interference (EMI) and deteriorating the service and work accuracy. Owing to the EMI effect, data leakage and insecurity have also been reported. Hence, it is necessary to control and manage the EM waves emitted by electronic gadgets to protect plants, animals, birds, humans, and the environment. Even though research on non-ionizing radiation began in the 1950s, the regulation of EM radiation exposure began after a long time. The International Non-ionizing Radiation Committee (INIRC) was established in 1977. Several organizations and regulation agencies have enforced regulations on human exposure to electromagnetic radiation at various frequencies. The American National Standards Institute (ANSI) published the NIR (non-ionizing radiation) exposure standard in 1982, which was handed over to IEEE. Furthermore, IEEE revised ANSI in 1991. In 1997, the U.S. published a regulation on cell phone radiation, and in 1998, the International Commission on Non-Ionizing Radiation Protection (ICNIRP, 1998) was provided (Gandhi et al., Citation2012).
2. EM wave attenuation mechanism
EM wave shielding has been a significant aspect of electronic system design that aims to prevent electromagnetic radiation from interfering with proper functioning. The basic mechanisms and theories of EMI shielding that play a crucial role in minimizing the effect of EMI are reflection, absorption, multiple reflections, and transmission. The conductive nature of the shielding material leads to the reflection of the EM wave incident on the material. Also, the permittivity (ε), angle of incidence, frequency of the EM wave incident on the material(), impedance mismatch, polarization state of the incident wave, and surface roughness can result in reflection loss. The reflection of the EM wave increases with the increase in electrical conductivity(
) of the shielding material upon which the EM wave incident. The absorption loss in the shielding materials is due to factors such as material composition,
magnetic permeability
ε, the thickness of the material,
material roughness, temperature, and multilayer configurations. Overall,
ε, and
are the significant influencers of the reflection and absorption loss.
The attenuation occurs by reflection (), absorption (
), and multiple reflection (
). An EM wave is incident on the shielding material; part of the wave is reflected, and part of the wave enters the material. Depending on the internal structure and properties of the material, waves entering the material may undergo multiple reflections and absorptions. The EM waves remaining in the material may pass through the material. Therefore, the total shielding effectiveness (
) is the sum of the three attenuation modes, as shown in EquationEq. (1)
(1)
(1) and is measured in decibels (dB) (González et al., Citation2018; Kondawar & Modak, Citation2020; Wilson et al., Citation2020). The
must be greater than 15 dB for commercial applications.
(1)
(1)
The should be lower; otherwise, the reflected wave interferes with the surrounding equipment and creates EMI.
The EM wave propagation in the shielding material along the thickness direction and the EM wave attenuation mechanism in the shielding material is illustrated in . The thickness at which the incident EM wave field strength decreased by 37% is called the skin depth From EquationEq. (2)
(2)
(2) , the
measures how deeply an EM wave penetrates into the shielding material. The
is inversely proportional to the square root of the frequency of the incident EM wave for a given material (constant µ and σ). The skin depth decreases with increasing frequency, so that the penetrated EM wave attenuates rapidly at higher frequencies.
(2)
(2)
Figure 2. EM Wave motion in a shielding material (Ganguly et al., Citation2018).

3. EM wave shielding in aircraft
Metals are excellent EM wave shielding materials. However, metal shielding has limitations, such as high density and high cost, which are susceptible to environmental degradation (Vo. Klemperer & Maharaj, Citation2009). Owing to these disadvantages, most metals, such as aluminum and ferrous alloys, are replaced by carbon fiber-reinforced polymer (CFRP) composites in aircraft. However, CFRP composites have attractive structural properties and low density, but one of the chief disadvantages is the lack of electromagnetic shielding capability owing to the non-conductive polymer matrix. However, modern aircraft have fly by-wire systems, and such aircraft contain more digital control systems. In addition, high-performance aircraft cockpits consist of more automated and digital navigation systems. Owing to the aforementioned advancements in aircraft, electromagnetic interference is a concern in the aviation industry (Schlechter, Citation2022). The EMI in an airplane can be classified into three categories. EMI due to (i) onboard systems, (ii) passenger carry-on electronic devices, and (iii) external sources, such as lightning strikes, high-intensity radiated fields (HIRF), and electromagnetic pulses. Because of the above sequences of EMI, even though CFRP is an excellent alternative to metals in aircraft, it is necessary to incorporate its shielding ability. Researchers have demonstrated the viability of CFRP composite materials for use as EMI shielding structures by adding fillers that enhance the conductivity and shielding effectiveness (SE) (Vo. Klemperer & Maharaj, Citation2009).
Generally, FRP composites are suitable for structural applications in aircrafts. The use of CFRP composites in aircraft is increasing. The typical structures, manufacturing methods, and applications of CFRP composites used in aircraft are listed in (Dutton et al., Citation2004). These CFRP parts require the ability to shield from EM radiation and have good structural properties. Therefore, research on EM radiation-shielding CFRP composites is essential. Also to enhance the structural properties in recent research found both metal and carbon fillers are used as secondary reinforcements and developed hybrid CFRP composites. It was reported in the literature that the quanity and type of filler addition to CFRP composites influence on structural properties (Agnihotri et al., Citation2022). Soliman et al. (Citation2012) demonstrated addition of MWCNTs enhanced the CFRP composites tension and in-plane shear strength. Rawat & Singh (Citation2016) showed addition of MWCNTs improved CFRPs flexural properties. Also MWCNTs influence on EM wave shileiding effectiveness of CFRP composites.
Table 1. Typical CFRP aerospace components are formed by several techniques (Dutton et al., 2014).
In addition, wearable materials are prepared using EM radiation shielding fabrics for people continuously interacting with EM radiation systems in avionics. Wearable EM shielding materials must be flexible, lightweight, and strong, and can absorb or reflect EM radiation incident on them. For this purpose, researchers have developed carbon-fabric-based wearable materials for EM shielding products such as gloves, coats, caps, and curtains.
4. Overview of manufacturing methods of CFRP composites for EM shielding materials
A flow chart followed by various researchers manufacturing CFRP composites for EM shielding applications is shown in . The carbon fabric should be cleaned using a suitable solvent to remove sizing chemicals and amorphous carbon loosely attached to the fiber. The sizing materials coated on the carbon fabric to facilitate fiber flow in the weaving machine should also be removed using this process. The pre-cleaning process is most important for obtaining an exemplary interface of secondary reinforcements, such as fillers, and coating on the fiber surface to enhance the EM shielding and structural properties of CFRP composites. The cleaning process is carried out by simply immersing the carbon fabric in a chemical solvent, such as acetone, for a set duration or cleaning by mechanical force using ultrasonication of the immersed fabric in liquid solvents.
Furthermore, CFRP composites were manufactured using cleaned CF through a two-stage process. In the first stage, the CF was modified by surface engineering. The surface of the carbon fiber was treated to create an activated surface. This process enhances the interface between the carbon fabric and the material deposited on the surface. Furthermore, electroless plating, physical vapor deposition, chemical vapor deposition, and metalizing were per formed to enhance the conductivity and magnetic permeability of the carbon fabric. In addition, a few researchers followed the filler dispersion to a suitable resin by the magnetic stirrer/ultrasonication/three-roll mill method, and the resultant filler mixed matrix was coated onto the carbon fabric by spray coating, immersion coating, or brush coating. The aforementioned process, also called the treatment of fiber or surface engineering of fibers, is essential to enhance the conductivity and magnetic permeability, in addition to the structural properties of CFRP EM shielding composites. The details of the process carried out by various researchers are discussed in the relevant sections of this article.
After the primary process, the surface-engineered CF is reinforced in a suitable matrix by any combination of the following methods: Vacuum-assisted resin transfer molding (VARTM), vacuum assisted resin infusion (VARI), Hand-lay-up method, and hot compression molding, to obtain CFRP EM wave shielding materials (Cecen & Sarikanat, Citation2008; Halimi et al., Citation2013; Menta et al., Citation2013; Xia et al., Citation2015a, Citation2015b).
5. Manufacturing and performance of CFRP EM shielding composites
Metal particles and metal oxide fillers, such as Nickel (Ni), Nickel ferrite (NiFe2O4), Ag, Titanium oxide (TiO2), CF, and Co (Cobalt), are used as secondary reinforcements with thermoplastic and thermoset matrices in CFRP composites to enhance EM wave shielding. In addition, the above-mentioned fillers are arranged on CF by dip coating, electroless plating, spray coating, and brush coating. Carbon-based fillers, such as carbon nanotubes (CNT), graphene oxide (GO), reduced graphene oxide (rGO), carbon black (CB), and short carbon fibers (SCF), are used as fillers in various types of CFRP composites to enhance the shielding effectiveness. Some studies have demonstrated the combination of carbonaceous and metal/metal oxide fillers to achieve tailored EM wave shielding, electrical conductivity, and structural properties. The properties of the resultant CFRP composite depend on the type of filler, aspect ratio, physical geometry of the filler, and intrinsic properties, such as conductivity, dielectric constant, and magnetic properties (Joshi et al., Citation2013; Thomassin et al., Citation2013). In addition, SE is also influenced by the fiber direction in the carbon fabric (Unidirectional, Bidirectional), the number of plies in the CF laminates, the architecture of multilayer laminates, and the material and type of interface nanomaterial layers used in the laminates. Hence, in this study, CFRP EM wave shielding composites were classified as (i) CFRP with metal and metal oxide filler composites, (ii) CFRP with carbonaceous filler composites, and (iii) wearable, flexible, and ultra-lightweight EM wave shielding composite fabric/films.
5.1. CFRP with metal and metal oxide fillers composites
Structural properties and EM shielding abilities are essential for structural EM shielding applications in aviation. In this regard, Zhu et al. (Citation2021) developed CFRP composites using the vacuum-assisted resin infusion (VARI) method with epoxy (E) as a matrix to obtain both mechanical and electromagnetic shielding effectiveness (EMSE). Initially, the sizing agents on the CF were removed by washing with acetone through sequential Soxhlet extraction at 60 °C for 2 h. Furthermore, the cleaned CF were activated using an activation solution under ultrasonic vibration conditions. The activated CF were placed in an electroless plating solution. Finally, the Ni-coated CF (CF-Ni) was washed with deionized (DI) water and dried in a vacuum oven at 80 °C for 12 h. They found that nickel (Ni) deposited by electroless plating on carbon fabric (CF) increased the conductivity and shielding effectiveness. Further, to increase the interaction between the electroless-plated Ni layer and epoxy, the samples were treated by self-polymerization of dopamine (PDA) before VARI molding. This led to the formation of covalent bonds between the interface materials and enhanced their structural properties. The surface treatment process of CF and the manufacturing process of CF-Ni composites are shown in . The PDA concentration adversely affected SE, but this effect was not significant. Owing to Ni deposition, the developed composites exhibited an EMSE of approximately 31 dB and were 53.5% higher than that of neat CF/E composites.
Figure 4. Ni-CF/E composite manufacturing steps (Zhu et al., Citation2021).

Kim et al. (Citation2017) also developed Ni-plated carbon fabric using an electroless method, further developed CF-Ni/E composites, and investigated the influence of the post-heat treatment temperature on SE and conductivity. They performed a post-heat treatment of Ni layers on CF from to 500-800 °C for approximately 30 min. The CF-Ni/E composites were developed using a hot press at 160 °C and 10 MPa for 60 min using a vacuum bagging method. They reported that the purity and crystallinity of the Ni layers on carbon fiber surfaces were improved by post-heat treatment. In addition, owing to the post-heat treatment, the porous structure formed in the Ni layer creates multiple reflections of EM waves, which leads to an improved EM wave shielding performance.
The laminates developed by 3-D carbon fabric/mat have specific requirements in avionics owing to their higher strength in the thickness direction compared to laminates made of 2D and unidirectional fabrics. Few researchers have investigated the EM shielding performance of 3D-fabric laminates. Magisetty et al. (Citation2019) synthesized Ni-NiFe2O4 cermets using a one-pot solvothermal method. They prepared an ABS solution by dissolving Acrylonitrile butadiene styrene (ABS) in methyl acetate using a magnetic stirrer at 500 rpm and 35 °C for 60 min. Further, the Ni-NiFe2O4 cermet compound with 10–40 wt% was dissolved in the ABS solution by ultrasonication for 30 min. The resultant cement-dispersed ABS was applied onto the 3D-carbon mat using the doctor blade-coating technique. The specimens were prepared and treated for 24 h at 300 K and 1 bar pressure. It was noticed that 0.92 mm thickness, 40 wt. % of Ni-NiFe2O4/ABS nanocomposite sample showed EMSE of 61.85 dB at 12 GHz and 54.81 dB at 8.1 GHz with hydrophobic water-repellent characteristics. Hence, they reported that such nanocomposites could be used efficiently for EM wave shielding under moist and humid weather conditions.
High-precision and sensitive electronic instruments require ultra-high-performance electromagnetic-shielding materials. In this context, Jia et al. (Citation2019) developed PU-AgNW–silver nanowire (PU-AgNW)/CF using the solution-coating method. They initially prepared AgNW dispersed alcohol solution; further cleaned CF fabric was dipped in AgNW/alcohol solution for approximately 1 min and dried at 80 °C for 15 min. The dipping process was repeated several times to increase the concentration of AgNWs on CF. shows a schematic of the fabrication of the PU-AgNW/CFF fabric. They found that the tensile strength and modulus of AgNW/CF increased as a function of dip-cycles, and more than 100 dB SE was achieved, which was constant for the X-band frequency range. In addition, the reduction in the SE and electrical conductivity after repetitive folding was found to be insignificant. Hence, the authors recommend composites suitable for EM shielding in harsh environments.
Figure 5. Schematic for the fabrication of the PU-AgNW/CFF fabric (Jia et al., Citation2019).

Park et al. (Citation2020) developed Ag-coated flexible woven carbon fiber fabrics by manual spray coating and roller-to-roller (R2R) coating. R2R spray coating was found to be an economical, promising, and scalable approach for manufacturing Ag-coated CF with good EM shielding capability. The shielding effectiveness increased as a function of Ag content in the carbon fabric. The double-layer and triple-layer Ag-ink coats on CF led to absorption-dominated SE and increased Ag content. However, the double- and triple-coated fabrics showed 11 to 14 dB reflection loss, and a more significant number of coated layers on the carbon fabric is beneficial.
Stupar et al. (Citation2022) developed a composite fabric by metalizing a silver conductive complex solution on CF. They followed a three-stage process to create silver-metalized CF: (i) synthesis of silver conductive complex solution further, (ii) coating of the silver conductive complex onto the CF surface, and (iii) sintering of the silver on CF. Three types of composite silver-metalized CF were developed by taking different annealing cycles as one, three, and five cycles and comparing the result with neat CF. They reported that the SE of neat CF is lower than the commercial requirement, and after the first annealing cycle, it meets the commercial requirement. They found that SE and conductivity increased with an increase in the number of annealing cycles. The silver-metallized CF with five annealing cycles showed approximately 50 dB in the X-band frequency range. In addition, owing to silver deposition on the CF, the mechanical properties improved. Hence, the modified CF with silver metallization can be recommended for designing warfare equipment.
Merizgui et al. (Citation2022) used ball-milled TiO2 and Co nanoparticles with diameters of 1 µm and 3 µm, respectively, and prepared films using the fluid diglycidyl ether of bisphenol-A-type epoxy. The ball-milling process of TiO2 and Co nanoparticles was carried out for 18 h with a powder-to-ball ratio of 1:15. Furthermore, hybrid composite laminates consisting of alternate layers of CF/with the addition of titanium dioxide (TiO2) and cobalt (Co) nanoparticle epoxy films were fabricated. They reported that the electrical conductivity of the hybrid laminates increased with increasing carbon fiber content in all configurations. In addition, the SE increased with the frequency from the E band to the J band. A similar trend was observed for the increased carbon fiber content. They concluded that the alternating layers in the shielding laminates effectively enhanced the SE.
CF-reinforced polyetheretherketone (PEEK) is an attractive aviation material owing to its high strength, stiffness, thermal stability, and biocompatibility. However, these composites have limitations such as poor wettability between CF and PEEK. Hence, in this direction, Yuan et al. (Citation2021) immersed CF into the Soxhlet extractor, added enough acetone, and desized at 70 °C for about 48 h. Further desized CF was washed with distilled water, dried under vacuum at 50–60 °C for 10 h, and immersed in 5.00 wt% potassium permanganate solution at 70 °C for 10 min. The oxidized CF were washed with distilled water and dried under vacuum at 60 °C for 5 h. After cleaning and activation, the CF surface was coated with polyamide-imide (PAI) and two-dimensional nanostructured Ti3C2Tx MXene. Finally, hot-compression molding was used to develop a hybrid CF composite. They reported that owing to the sizing of CF by PAI, the flexural strength, modulus, and interlaminar shear strength improved by 52.49%, 112.51%, and 74.47%, respectively. In addition, EM shielding and conductivity increased owing to the addition of MXene nanosheets. shows the schematic diagram of this process.
5.2. CFRP with carbonaceous filler composites
Carbon-based materials, such as single-wall carbon nanotubes (SWCNT), multi-walled carbon nanotubes (MWCNT), graphene oxide(GO), reduced graphene oxide(rGO), carbon black (CB), and carbon nanofiber (CNF)/short carbon nanofibers (SCNF), are used as fillers. Carbon-based nanomaterials can also absorb microwave radiation. Therefore, absorption-dominated shielding can be achieved in carbon-based polymer nanocomposites. In addition, carbon-based filler-reinforced polymer nanocomposites exhibit good mechanical properties, low specific gravity, and resistance to various external conditions (Jiang et al., Citation2019; Pawar et al., Citation2016; Sankaran et al., Citation2018). Hence, these composites are used as microwave radiation absorbing materials. Lee et al. (Citation2022) developed flexible prepregs using SWCNT and graphene oxide ink coated on polyester fabric (PF) impregnated with an epoxy matrix. shows a schematic representation of the preparation process of the hybrid carbon ink‑loaded polyester nonwoven fabric/epoxy composite (S‑x/G/PF/Ep).
Figure 7. Schematic representation of the preparation process of the hybrid carbon ink‑loaded polyester nonwoven fabric/epoxy composites (S‑x/G/PF/Ep).

The prepregs were manufactured with various SWCNT concentrations (wt. %) and graphene. Furthermore, the prepared prepregs were used to manufacture G/PF/E, S-0.5/G/PF/E, S-1/G/PF/E, S-2/G/PF/E, and S-4/G/PF/E composites via vacuum-assisted molding. They noticed that the electrical conductivity and SE increased as a function of the SWCNT concentration up to 2 wt. % and found a reduction in conductivity and SE due to further increased concentration of SWCNT in the composite. This is due to the agglomeration of SWCNTs at higher concentrations ( In addition, the absorption-dominated SE due to SWCNTs in the composites increased the absorption SE, and the reflection mode of the SE decreased as a function of SWCNT concentration in the composites up to 2 wt %. It is found that 2 Wt.% SWCNT loaded composite showed approximately 40 dB, which is the highest among other composites tested by this research.
Yesmin & Chalivendra (Citation2021) fabricated GF(Glass fiber/SCF/MWCNT/E and GF/SCF/MWCNT//E composites using vacuum-assisted molding. The SCFs were oriented in the material thickness direction by the electro-flocking method, as shown in . They used three types of carbon fibers with lengths of 80 µm, 150 µm, and 350 µm. They maintained 1000 and 2000 SCF per
In addition, the varied
concentration was varied 0.5 and 1 wt. %. In their investigation of the effects of CNT, SCF, and
nanoparticles on the electromagnetic shielding effectiveness in the X-band frequency range, they discovered that
nanoparticles had the most significant influence on SE. The absorption significantly influenced the total SE of all the composites. Composites with
nanoparticles (1.0 wt. %) and carbon fibers (350 µm long with fiber density of 2000 fibers/
) revealed the highest SE and the dominance of absorption. The fiber density per unit area increase did not show any significant influence on the SE in composites with no
nanoparticles and with 0.5 wt. % of
nanoparticles. The 1.0 wt.% of
nanoparticles having a higher fiber density of 2000 fibers/
composites of carbon fiber lengths 80 µm and 150 µm showed favorable improvements in total SE values and SE values due to absorption as compared to those of 1000 fibers/
Ucar et al. (Citation2018) investigated graphene oxide fibers, reduced graphene oxide, and MWCNTS as fillers in CF fabric-reinforced epoxy. Graphene oxide fibers and reduced graphene oxide have a highly rough surface that causes multiple reflections, resulting in a decline in SE. However, the high conductivity of the 15 wt. % loadings showed 32 dB SE.
Singh et al. (Citation2012) developed neat carbon fabric epoxy composites and MWCNT/carbon fabric epoxy composites by applying the compression molding method. They maintained a reinforcement-to-matrix ratio of 30:70 by volume for all samples. They also considered MWCNT volume fractions of 1,2,3 and 4%. They found that as the content of MWCNT increased in the composite, the skin depth decreased and the total SE increased. They also observed that higher MWCNT-reinforced composites exhibited more absorption-dominated SE. The systematic preforms used for composite manufacturing increased the mechanical properties, particularly the interlaminar shear strength and conductivity of the composites. Ghosh et al. (Citation2023) developed light weight thermoplastic films by incorporating the CNT and carbon black (CB) in polystyrene/ethylene-co-methyl acrylate blend. They reported the hybrid filler (CNT & CB) in the thermoplastic elastomeric blend developed interconnected double percolated conductive path in the composite film and it improved the electrical conductivity, dielectric, thermal properties and EMI shielding effectiveness.
5.3. Wearable, flexible, ultra-lightweight EM wave shielding composite fabric/films
Wearable EM shielding materials must have structural flexibility and air permeability, be lightweight, and be self-cleaning. Lu et al. (Citation2020) developed a superhydrophobic, highly conductive, and flexible CEF-NF/PDA/Ag fabric with stable EM shielding in the X-band frequency range. A bioinspired electroless silver plating strategy and one-step electrodeposition method were utilized to prepare the fabric. It exhibited up to 101.27 dB of SE, protection against oxidation, and superhydrophobicity. They also reported that the developed fabric has good potential for aerospace wearable EM shielding applications.
Gamage (Citation2017) developed a series of MWCNT-coated nonwoven carbon fabrics via a facile dip-coating process. They reported that MWCNTs coated on the carbon fabric filled the pores in the carbon fabric and achieved a conductivity of 16.42 S/cm and an EM SE of 37 dB at 3 GHz in a single layer. Furthermore, it was enhanced to 68 dB at 2.7 GHz in a double layer. They also noticed an improvement in the tensile strength due to the addition of MWCNT and thermal stabilization. The developed MWCNT/carbon fabric is lightweight and flexible, and can be used in aerospace and automobile applications.
Xing et al. (Citation2018) developed a highly flexible ultrathin carbon fabric/polycarbonate (CF/PC) film and carbon fabric/polycarbonate/nickel (CF/PC/Ni) films with various concentrations of Ni for EM shielding applications. They reported that electroless Ni plating improved the SE of the films, and it increased with Ni plating time. Ni plating for 40 min at a thickness of 310 mm showed the highest EMI-SE of 72.7 dB. In addition, the developed Ni-plated CF/PC films showed good mechanical strength, thermal stability, and flexibility.
6. Influencing parameter on EM wave shielding effectiveness of multilayer CF laminated composites
The neat CFRP composites (without filler) exhibited EM wave shielding properties owing to the carbon fibers in the composite. Gupta et al. (Citation2021) developed CF fabric/epoxy laminates of thickness 2.3 mm using ten numbers of unidirectional CF prepregs of each 0.21 mm thickness by hot compression molding followed by a post-curing method. They claimed that CF laminates have a propensity to absorb electromagnetic waves at X-band frequencies. However, they observed higher reflection. Various researchers have studied different factors in manufacturing CFRP EM shielding materials, as shown in , to increase the absorption mode of shielding and enhance shielding effectiveness.
Figure 9. Factors influencing EM wave shielding effectiveness and structure properties of CFRP multilayer laminates.

The direction and orientation of the fiber, layer architecture, interface layer, and number of plies in the multilayer structure influenced the EM wave shielding of the CF composites. Hong et al. (Citation2021) investigated the impact of layup configuration, carbon fiber type, number of layers, and fiber orientation on the EM shielding of CFRP composite laminates. They found that carbon fiber prepreg stacked composite laminates have anisotropic conductivity and dielectric characteristics, leading to anisotropic electromagnetic properties. They reported that the EM SE was affected by the orientation of the fiber, and the four-ply CFRP laminate () with three cross-layers showed 55% improved shielding effectiveness compared to UDCFRP (
) composite laminates. Aripin et al. (Citation2020) studied the mechanical properties and EM shielding effectiveness of unidirectional carbon fabric (UDCF) tapes. Carbon fabric-reinforced thermoplastic (CFRTP) composites were prepared using polyamide-6 as the matrix and reinforcement UDCF (made of PAN-based T700SC continuous carbon fiber and randomly chopped carbon fiber). The influence of the carbon fiber direction on the mechanical properties and SE was studied by considering UDCF tape with different layer structures in the 0°, 90°, and ±45° directions. They investigated three different layer configurations: unidirectional [
bidirectional
and quasi-isotropic
It has been reported that the UD layer configuration shows superior flexural strength compared to other configurations. However, the chopped type showed an improvement in the modulus. The SE in all configurations is absorption-dominated, and it is higher for the cross-ply configuration than UD. The randomly chopped CFRTP exhibited a lower EMSE. Coskun (Citation2022) developed bidirectional and unidirectional carbon fiber fabric-reinforced epoxy composites. It was reported that the EMSE was very high when the orientation angle of the carbon fiber was
as compared to
Hence, they demonstrated that bidirectional carbon fabric-reinforced composite laminates show higher EMSE than unidirectional carbon fabric composites. In addition, the number of plies has a smaller effect than the orientation angle. Bedi et al. (Citation2018) investigated the effect of carbon nanotubes (CNT) grafting on the wetting behavior and the mechanical properties of carbon fiber/epoxy composites. They found that CNT grafting improves the interfacial shear strength and mechanical response in the carbon fiber/epoxy composites. They observed that CNT grafting has no significant effect on the mechanical properties of the carbon fibers/polyester composites. They concluded that choosing the polymer matrix is essential for enhancing the wettability for CNT grafting and selecting the fiber orientation is another factor in improving the properties of the composites.
Ma et al. (Citation2022) initially prepared polyacrylonitrile (PAN) nanofiber (NF) sheets by electrospinning a PAN precursor solution at 25 °C and 39% relative humidity (RH). The PAN NF sheets were then carbonized and converted into carbon NF sheets. The two developed carbon NF sheets were placed in the upper and bottom plies of the UD carbon fiber prepregs, called a preform. Furthermore, the performance was subjected to a vacuum bagging technique, and laminates designated as C-P-P-C were developed. They also prepared laminates consisting of two plies of carbon fiber prepregs without a carbon NF sheet, designated as P-P. It was reported that the SE of the C-P-P-C laminates improved by 35% compared to that of the P-P laminates. This was attributed to most of the incident EM waves in the case of the C-P-P-C laminates interacting with the carbon NFs at the bottom and top sides of the laminates and increased attenuation by absorption. They also observed a small difference in the interlaminar shear strength of the laminates. Panapoy et al. (Citation2008) prepared precursor solution of polyacrylonitrile (PAN) and obtained NFs through the electrospinning technique by maintaining the voltage at 17 kV, nozzle tip to collector distance at 15 cm and a flow rate of 2.17 ml/h. They observed that the fibers had a smooth structure with a fiber diameter of 370 nm. The as spun PAN NFs were heat treated and carbonized (800 - 1000 °C) to form carbon NFs. They found that the diameter of the NFs heat treated at 1000 °C had lower diameter of about 200 nm. The FTIR spectra showed the disappearance of nitrile bond in the carbonized NFs. The Raman spectroscopy analysis, revealed that carbonizing the NFs at higher temperatures (1000 °C) resulted in a reduction of the intensity ratio of D and G peaks, indicating a higher graphitic structure. The XRD patterns showed the formation of graphite structure in carbon NFs. Also, due to the semiconducting nature of the NF, an increase in the electrical conductivity was observed as the temperature increased. Kim et al. (Citation2021). studied the properties of composite membranes made of ultrathin and lightweight multiwalled carbon nanotube (MWCNT) buckypaper and electrospun PAN NFs. This was achieved through ES, calcination, and vacuum filtration. The ES was carried out with a feed rate of 1 mL/h, a voltage of 12 kV was applied and the distance between the needle tip to the collector was maintained at 15 cm. The as-spun NFs were calcinated at various temperatures (40, 70, 100, and 130 °C). Simillarly, the The bucky paper/nanofiber (BP/NF) composite membranes were fabricated by suspension vacuum filtration. The NFs had an average diameter of 800 nm with bead free structures. The NFs calcined at 130 °C was densely packed composite membrane showed superior mechanical strength of 19 MPa and high surface energy with excellent adhesion for the MWCNT. This resulted in the enhancement of the interfacial interaction between the BP and the NF surfaces. They observed that the BP/NF calcined at 130 °C had an electrical conductivity, superior EMI SE, and EMI specific shielding effectiveness of 6.177 S/cm, 23.3 dB, and 13 734 dB cm2/g. They concluded that these materials can be applicable for practical, and high performance EMI shielding applications such as aerospace, military, smart, wearable, and flexible electronics.
Jou (Citation2004) experimentally and theoretically investigated the influence of weave type, number of plies, and overlap angle between overlap plies of continuous carbon fiber epoxy composites on SE. They reported that the SE of woven carbon-fiber epoxy composites was higher than that of discontinuous carbon-fiber epoxy composites. They reported that single, double, and triple plain and balanced-twill woven carbon fiber epoxy composites showed 50 dB, 60 dB, and 70 dB in a 3 GHz range frequency. An SE of more than 100 dB was obtained for the triple plain-weave composite plates.
Research gaps, challenges, future scope, and conclusion
The EM wave attenuation in a shielding material is caused by absorption, reflection, and multiple reflections. The best shielding material should have EM shielding by absorption and multiple reflections, rather than reflection. This is because reflected EM waves tend to interfere with EM waves emitted by neighboring devices and deteriorate their intended function.
Metal fillers such as Ni, Fe2O4, Ag nanowire and nanoparticles, TiO2, and Co are incorporated in CFRP composites by mixing with the matrix, coating on CF, or coating on the CFRP laminates to enhance SE and electrical conductivity.
Most carbonaceous fillers such as SWCNT, MWCNT, graphene oxide, reduced graphene oxide, short carbon fiber, and MXene nanosheets have been incorporated into CFRP composites by researchers through various methods such as coating on CF, growing carbon nanomaterials on CF substrates through in-situ processes, and using carbonaceous fillers as secondary reinforcements in the matrix. They reported that the structural property and SE of CFRP enhanced by filler addition also found absorption-dominant SE in carbonaceous filler-reinforced CFRP composites.
Most studies have used plain view-type CF fabrics to develop composites for EM wave shielding. Multi-layered structures, various architectures of layers, and nanofilm interfaces between CF plies have also been studied. The direction of the fiber orientation in EM radiation propagation also influences the SE. This composite type can increase multiple reflections inside the composite material and enhance absorption-dominated shielding. The 3-D CF are used in the aerospace sector owing to their suitable structural properties in the thickness direction. Few researchers have conducted electromagnetic shielding studies on 3D CF hybrid composites.
The summary of the SE and conductivity achieved by various researchers, material combinations used, fiber/plies architecture, and fabrication method used to develop the composites are listed in . The SE achieved by various researchers for CF RP composites is shown in the bar chart in . It was observed that few researchers achieved SE in the range of 100 dB in the X-band frequency with attractive mechanical properties in lightweight, highly flexible, and ultra-thin materials. Hence, there is a large scope to develop such materials using a cost-effective method with high shielding effectiveness.
Ultra-lightweight, highly flexible, air-permeable, water-repellent, and resistant to harness are essential properties of wearable EM shielding CF-based composites for various avionic applications. It can also be used as the top skin and components of aircraft and aerospace vehicles. Hence, this is an attractive area with scope for future research.
Vacuum-assisted resin transfer molding (VARTM) and vacuum-assisted resin infusion (VARI) are well-established manufacturing techniques for producing high-performance CFRP composites. VARTM is cheap and reduces the emission of volatile organic compounds compared to hot compression molding and hand layup methods. In addition, using the VARTM method, one can produce CFRP composites with improved mechanical and physical properties compared to hand layup and hot compression molding.
Table 2. The manufacturing method, material combination, conductivity, and shielding effectiveness of CFRP composites.
Authors’ contributions
Suresha R: Literature survey and original draft preparation. B Shivamurthy: Conceptualization, supervision, administration. Sachidananda H K: Co-supervision and reviewing. Niranjan N Prabhu: Literature survey, editing. Prashant M Prabhu: Literature survey, editing. Rajiv Selvam: Reviewing.
Acknowledgments
The authors gratefully acknowledge support provided by the Manipal Academy of Higher Education.
Disclosure statement
No potential conflict of interest was reported by the author (s).
Data availability statement
All data used for this research is included in this manuscript.
Additional information
Funding
Notes on contributors
Suresha R.
Mr. Suresha R is pursuing his Ph.D. in Carbon Fabric Reinforced Hybrid Structural Composites for Electromagnetic Radiation Shielding at the Manipal Academy of Higher Education, Manipal, India. He received a bachelor’s degree in Electronics Engineering in the year 1996 from Bangalore University, Karnataka, India, and a Master of Technology in Power Electronics in the year 2007 from Visveswaraya Technological University (VTU), Belgaum, Karnataka, India. He has 26 years of Teaching Experience. He has guided more than 10 Post Graduation Projects. His research interests are in the field of composite materials. He currently holds the position of Assistant Professor – Selection Grade at Manipal Academy of Higher Education, located at the School of Engineering and IT in Dubai Campus.
B. Shivamurthy
Dr. B Shivamurthy, received the B.E. degree in mechanical engineering from Mysore University, India, in 1991, M. Sc. Engineering by research degree in mechanical engineering from Visvesvaraya Technological University, Belagavi, India in 2008. He obtained Ph. D in Metallurgical & Materials Engineering from National Institute of Technology Karnataka in 2014. He is currently working as Professor at Manipal Institute of Technology, Manipal Academy of Higher Education, Manipal, India. From 1991 to 2001, he was worked with the polymer engineering Industries in production, R&D in various sectors such as polyester films, thermoset & thermoplastic composites for various applications. He also executed selection and installation of plant and machinery for the same. Further 2001 till date he is working as an Engineering faculty and researcher in India. He also worked as program coordinator at Manipal School of Engineering and IT at Dubai. His research interest includes polymer composites, Nano hybrid composites for electronics, biomedical, and engineering sectors. Dr. B. Shivamurthy published research articles in the field of electronic materials, wearresistant polymers, structure properties of various Nano hybrid polymer composites, fire retardant materials, EMI Shielding, and impact-resistant materials.
Sachidananda H. K.
Dr. Sachidananda currently holds the positions of Assistant Registrar and Associate Professor at Manipal Academy of Higher Education, located at the School of Engineering and IT in Dubai. With a robust background spanning 25 years in the education sector, he earned his doctoral degree from Manipal Institute of Technology in Manipal, India. Dr. Sachidananda has demonstrated his scholarly prowess by authoring over 40 publications in international journals and conferences. His contributions extend beyond the written word; he has been recognized as a session chair at international conferences in the UAE. Notable accolades include receiving the Best Paper Presentation Award at the ICMT SET conference in Dubai and the Best Poster Presentation Award at Manipal University, India. Dr. Sachidananda’s research pursuits delve into areas such as Machine Design, Altered Tooth-Sum Gearing, Optimization through Taguchi and Grey Relation Analysis, Artificial Neural Networks (ANN), and various aspects of manufacturing processes.
Niranjan N. Prabhu
Mr. Niranjan N Prabhu is a research scholar in the department of Mechanical & Industrial Engineering at Manipal Institute of Technology, Manipal Academy of Higher Education, Manipal. He received his B.E degree in Mechanical Engineering in the year 2013, and MTech in Design Engineering in the year 2016. He has worked as an Automotive Design Engineer from 2017 to 2020 in Pune. His research interests include electrospinning, and gas sensors.
Prashant M. Prabhu
Mr. Prashant M Prabhu is pursuing his Ph.D. in sustainable electromagnetic interference shielding materials at the Manipal Academy of Higher Education (MAHE), Manipal, India. He received a bachelor’s degree in Electronics and Communication Engineering in the year 1998 from Karnataka University, Dharwad, India, and a Masters in Digital electronics and Communication in the year 2005 from Visvesvaraya Technological University, Belagavi, India. He has 24 years of teaching experience. He has guided more than 10 post-graduation student projects. His research interests are in the field of composite materials. He has published research articles in the field of EMI shielding materials.
Rajiv Selvam
Dr. Rajiv Selvam currently serves as Deputy Chairperson-School of Engineering and IT & Associate Professor at the Department of Mechanical Engineering, Manipal Academy of Higher Education, Dubai. He acquired a graduate (B.E.) in Mechanical Engineering and a post-graduate (M.E.) in Engineering Design. He completed his doctoral work (Ph.D.) at the College of Engineering, Guindy, Anna University, Chennai. In addition, he also has been employed as a Visiting Research Scholar with the Mechanical Engineering Department, College of Engineering in American University of Sharjah. Dr. Rajiv Selvam has over 18 years of teaching experience, including nine years abroad. He has served as a visiting faculty member in leading institutes in the Sultanate of Oman, Morocco, and Ethiopia. To his credit, he has two patents and published more than 30 Research Papers in Scopus Indexed Journals. His areas of research include Electromagnetic Forming, Composite Materials, and Non-Conventional Machining Techniques. Dr. Rajiv Selvam is recognized and honored with many awards such as the Best Teacher Award, Academic Excellence Award, Best Project Award and Best Paper Award by Institutes/Professional Bodies of repute, for his contribution to the field of education. He has organized One International workshop, four International Conferences, one National Conference and many professional society events. He is also an Associate member of IMechE and a Member of Indian Society of Technical Education (ISTE).
References
- Agnihotri, S. N., Thakur, R. K., & Singh, K. K. (2022). Influence of nanoclay filler on mechanical properties of CFRP composites. Materials Today: Proceedings, 66, 1734–1738. https://doi.org/10.1016/j.matpr.2022.05.270
- Aripin, A. B., Nishi, M., Suzuki, K., & Hayakawa, K. (2020). Evaluation of the mechanical and electromagnetic shielding properties of carbon fiber reinforced thermoplastics sheet made of unidirectional tape. Materials Transactions, 61(2), 251–255. https://doi.org/10.2320/matertrans.MT-ML2019008
- Balodis, V., Brmelis, G., Kalvikis, K., Nikodemus, O., Tjarve, D., & Znotia, V. (1996). Does the Skrunda Radio Location Station diminish the radial growth of pine trees? Science of the Total Environment, 180(1), 57–64. https://doi.org/10.1016/0048-9697(95)04920-7
- Bedi, H. S., Padhee, S. S., & Agnihotri, P. K. (2018). Effect of carbon nanotube grafting on the wettability and average mechanical properties of carbon fiber/polymer multiscale composites. Polymer Composites. 39(S2), E1184–E1195. https://doi.org/10.1002/pc.24714
- Cecen, V., & Sarikanat, M. (2008). Experimental characterization of traditional composites manufactured by vacuum-assisted resin-transfer molding. Journal of Applied Polymer Science, 107(3), 1822–1830. https://doi.org/10.1002/app.27235
- Coskun, Y. (2022). The impact of orientation angle and number of layers on electromagnetic shielding characteristics of carbon fiber composites. Journal of Innovative Science and Engineering, 6(2), 190–200.
- Diem, E., Schwarz, C., Adlkofer, F., Jahn, O., & Rüdiger, H. (2005). Non-thermal DNA breakage by mobile-phone radiation (1800 MHz) in human fibroblasts and in transformed GFSH-R17 rat granulosa cells in vitro. Mutation Research, 583(2), 178–183. https://doi.org/10.1016/j.mrgentox.2005.03.006
- Dutton, S., Kelly, D., & Baker, A. (2004). Composite materials for aircraft structures (2nd ed.). https://doi.org/10.2514/4.861680
- Gamage, S. J. P. (2017). MWCNT coated free-standing carbon fiber fabric for enhanced performance in EMI shielding with a higher absolute EMI SE. Materials (Basel), 10(12), 1350. https://doi.org/10.3390/ma10121350
- Gandhi, O. P., Morgan, L. L., De Salles, A. A., Han, Y. Y., Herberman, R. B., & Davis, D. L. (2012). Exposure limits: The underestimation of absorbed cell phone radiation, especially in children. Electromagnetic Biology and Medicine, 31(1), 34–51. https://doi.org/10.3109/15368378.2011.622827
- Ganguly, S., Bhawal, P., Ravindren, R., & Das, N. C. (2018). Polymer nanocomposites for electromagnetic interference shielding: A review. Journal of Nanoscience and Nanotechnology, 18(11), 7641–7669. https://doi.org/10.1166/jnn.2018.15828
- Ghosh, S. K., Nath, K., Nath Chowdhury, S., Paul, S., Ghosh, T., Katheria, A., Das, P., & Das, N. C. (2023). Combination effect of functionalized high aspect ratio carbonaceous nanofillers and carbon black on electrical, thermal conductivity, dielectric and EMI shielding behavior of co-continuous thermoplastic elastomeric blend composite films. Chemical Engineering Journal Advances, 15, 100505. https://doi.org/10.1016/j.ceja.2023.100505
- González, M., Pozuelo, J., & Baselga, J. (2018). Electromagnetic shielding materials in GHz range. Chemical Record (New York, N.Y.), 18(7–8), 1000–1009. https://doi.org/10.1002/tcr.201700066
- Gupta, H., Agnihotri, P. K., Basu, S., & Gupta, N. (2021). Electrical characterization of carbon fiber reinforced polymer composites [Paper presentation]. 2021 electrical insulation conference, EIC 2021. https://doi.org/10.1109/EIC49891.2021.9612275
- Halimi, F., Golzar, M., Asadi, P., & Beheshty, M. H. (2013). Core modifications of sandwich panels fabricated by vacuum-assisted resin transfer molding. Journal of Composite Materials. 47(15), 1853–1863. https://doi.org/10.1177/0021998312451763
- Hong, J., Xu, P., Xia, H., Xu, Z., & Ni, Q. Q. (2021). Electromagnetic interference shielding anisotropy enhanced by CFRP laminated structures. Composites Science and Technology. 203, 108616. https://doi.org/10.1016/j.compscitech.2020.108616
- Jagadeesh Chandra, R. B., Shivamurthy, B., Kulkarni, S. D., & Kumar, M. S. (2019). Hybrid polymer composites for EMI shielding application- a review. Materials Research Express, 6(8), 082008. https://doi.org/10.1088/2053-1591/aaff00
- Jia, L. C., Xu, L., Ren, F., Ren, P. G., Yan, D. X., & Li, Z. M. (2019). Stretchable and durable conductive fabric for ultrahigh performance electromagnetic interference shielding. Carbon N. Y, 144, 101–108. https://doi.org/10.1016/j.carbon.2018.12.034
- Jiang, D., Murugadoss, V., Wang, Y., Lin, J., Ding, T., Wang, Z., Shao, Q., Wang, C., Liu, H., Lu, N., Wei, R., Subramania, A., & Guo, Z. (2019). Electromagnetic Interference Shielding Polymers and Nanocomposites - A Review. Polymer Reviews, 59(2), 280–337. https://doi.org/10.1080/15583724.2018.1546737
- Joshi, A., Bajaj, A., Singh, R., Alegaonkar, P. S., Balasubramanian, K., & Datar, S. (2013). Graphene nanoribbon-PVA composite as EMI shielding material in the X band. Nanotechnology, 24(45), 455705. https://doi.org/10.1088/0957-4484/24/45/455705
- Jou, W. S. (2004). A novel structure of woven continuous-carbon fiber composites with high electromagnetic shielding. Journal of Electronic Materials, 33(3), 162–170. https://doi.org/10.1007/s11664-004-0175-x
- Kim, K. W., Han, W., Kim, B. S., Kim, B. J., & An, K. H. (2017). A study on EMI shielding enhancement behaviors of Ni-plated CFs-reinforced polymer matrix composites by post heat treatment. Applied Surface Science. 415, 55–60. https://doi.org/10.1016/j.apsusc.2017.01.108
- Kim, M., Kim, S., Seong, Y. C., Yang, K. H., & Choi, H. (2021). Multiwalled carbon nanotube buckypaper/polyacrylonitrile nanofiber composite membranes for electromagnetic interference shielding. ACS Applied Nano Materials, 4(1), 729–738. https://doi.org/10.1021/acsanm.0c03040
- Kondawar, S. B., & Modak, P. R. (2020). Theory of EMI shielding. Elsevier Inc. https://doi.org/10.1016/b978-0-12-817590-3.00002-6
- Lee, J. H., Kim, Y. S., Ru, H. J., Lee, S. Y., & Park, S. J. (2022). Highly flexible fabrics/epoxy composites with hybrid carbon nanofillers for absorption-dominated electromagnetic interference shielding. Nano-Micro Letters, 14(1), 188. https://doi.org/10.1007/s40820-022-00926-1
- Lu, L., Xie, Y., Mei, X., Yu, Y. X., Tang, Y., & Teh, K. S. (2020). Preparation of flexible carbon fiber fabrics with adjustable surface wettability for high-efficiency electromagnetic interference shielding. ACS Applied Materials & Interfaces, 12(43), 49030–49041. https://doi.org/10.1021/acsami.0c08868
- Ma, Y., Zhuang, Y., Li, C., Shen, X., & Zhang, L. (2022). Improving electromagnetic interference shielding while retaining mechanical properties of carbon fiber-based composites by introducing carbon nanofiber sheet into laminate structure. Polymers, 14(9), 1658. https://doi.org/10.3390/polym14091658
- Magisetty, R. P., Shukla, A., & Kandasubramanian, B. (2019). Terpolymer (ABS) cermet (Ni-NiFe2O4) hybrid nanocomposite engineered 3D-carbon fabric mat as a X-band electromagnetic interference shielding material. Materials Letters. 238, 214–217. https://doi.org/10.1016/j.matlet.2018.12.023
- Menta, V., Vuppalapati, R., Chandrashekhara, K., Schuman, T., & Sha, J. (2013). Elevated-temperature vacuum-assisted resin transfer molding process for high performance aerospace composites. Polymer International, 62(10), 1465–1476. https://doi.org/10.1002/pi.4444
- Merizgui, T., Prakash, V. R. A., Gaoui, B., & Sebaey, T. A. (2022). Microwave shielding performance of TiO2/Co/GF containing high structure carbon fiber alternate laminate composite. Journal of Materials Science: Materials in Electronics, 33(2), 934–949. https://doi.org/10.1007/s10854-021-07365-5
- Monselise, E. B. I., Levkovitz, A., Gottlieb, H. E., & Kost, D. (2011). Bioassay for assessing cell stress in the vicinity of radio-frequency irradiating antennas. Journal of Environmental Monitoring: JEM, 13(7), 1890–1896. https://doi.org/10.1039/c1em10031a
- Panapoy, M., Dankeaw, A., & Ksapabutr, B. (2008). Electrical conductivity of PAN - based carbon nanofibers prepared by electrospinning method. Thammasat International Journal of Science and Technology, 13, 11–17.
- Park, J., Hu, X., Torfeh, M., Okoroanyanwu, U., Arbabi, A., & Watkins, J. J. (2020). Exceptional electromagnetic shielding efficiency of silver coated carbon fiber fabrics: Via a roll-to-roll spray coating process. Journal of Materials Chemistry C, 8(32), 11070–11078. https://doi.org/10.1039/D0TC02048F
- Pawar, S. P., Biswas, S., Kar, G. P., & Bose, S. (2016). High frequency millimetre wave absorbers derived from polymeric nanocomposites. Polymer, 84, 398–419. https://doi.org/10.1016/j.polymer.2016.01.010
- Rawat, P., & Singh, K. K. (2016). A strategy for enhancing shear strength and bending strength of FRP laminate using MWCNTs. IOP Conference Series: Materials Science and Engineering, 149, 012105. https://doi.org/10.1088/1757-899X/149/1/012105
- Sankaran, S., Deshmukh, K., Ahamed, M. B., & Khadheer Pasha, S. K. (2018). Recent advances in electromagnetic interference shielding properties of metal and carbon filler reinforced flexible polymer composites: A review. Composites Part A: Applied Science and Manufacturing, 114, 49–71. https://doi.org/10.1016/j.compositesa.2018.08.006
- Schlechter, M. (2022). “No Title,” EMI: Materials and technologies – abstract. [Online]. http://www.electronics.ca/
- Singh, B. P., Choudhary, V., Saini, P., & Mathur, R. B. (2012). Designing of epoxy composites reinforced with carbon nanotubes grown carbon fiber fabric for improved electromagnetic interference shielding. AIP Advances, 2(2), 022151. https://doi.org/10.1063/1.4730043
- Soliman, E., Al-Haik, M., & Taha, M. R. (2012). On and off-axis tension behavior of fiber reinforced polymer composites incorporating multi-walled carbon nanotubes. Journal of Composite Materials. 46(14), 1661–1675. https://doi.org/10.1177/0021998311422456
- Stein, Y., Hänninen, O., Huttunen, P., Ahonen, M., & Ekman, R. (2015). Electromagnetic radiation - environmental indicators in our surroundings. Environmental indicators. 1011–1024. https://doi.org/10.1007/978-94-017-9499-2_56
- Stupar, S. L., Vuksanović, M. M., Mijin, D. Ž., Milanović, B. C., Joksimović, V. J., Barudžija, T. S., & Knežević, M. R. (2022). Multispectral electromagnetic shielding and mechanical properties of carbon fabrics reinforced by silver deposition. Materials Chemistry and Physics. 289, 126495. https://doi.org/10.1016/j.matchemphys.2022.126495
- Thomassin, J. M., Jérôme, C., Pardoen, T., Bailly, C., Huynen, I., & Detrembleur, C. (2013). Polymer/carbon based composites as electromagnetic interference (EMI) shielding materials. Materials Science and Engineering R: Reports, 74(7), 211–232. https://doi.org/10.1016/j.mser.2013.06.001
- Ucar, N., Kayaoğlu, B. K., Bilge, A., Gurel, G., Sencandan, P., & Paker, S. (2018). Electromagnetic shielding effectiveness of carbon fabric/epoxy composite with continuous graphene oxide fiber and multiwalled carbon nanotube. Journal of Composite Materials. 52(24), 3341–3350. https://doi.org/10.1177/0021998318765273
- Vo. Klemperer, C. J., & Maharaj, D. (2009). Composite electromagnetic interference shielding materials for aerospace applications. Composite Structures. 91(4), 467–472. https://doi.org/10.1016/j.compstruct.2009.04.013
- Wilson, R., George, G., & Joseph, K. (2020). An introduction to materials for potential EMI shielding applications. Status and future. Elsevier Inc. https://doi.org/10.1016/b978-0-12-817590-3.00001-4
- Xia, C., Shi, S. Q., & Cai, L. (2015a). Vacuum-assisted resin infusion (VARI) and hot pressing for CaCO3 nanoparticle treated kenaf fiber reinforced composites. Compos. Part B Eng, 78, 138–143. https://doi.org/10.1016/j.compositesb.2015.03.039
- Xia, C., Shi, S. Q., Cai, L., & Hua, J. (2015b). Property enhancement of kenaf fiber composites by means of vacuum-assisted resin transfer molding (VARTM). Holzforschung, 69(3), 307–312. https://doi.org/10.1515/hf-2014-0054
- Xing, D., Lu, L., Teh, K. S., Wan, Z., Xie, Y., & Tang, Y. (2018). Highly flexible and ultra-thin Ni-plated carbon-fabric/polycarbonate film for enhanced electromagnetic interference shielding. Carbon N. Y, 132, 32–41. https://doi.org/10.1016/j.carbon.2018.02.001
- Yesmin, N., & Chalivendra, V. (2021). Article electromagnetic shielding effectiveness of glass fiber/epoxy laminated composites with multi-scale reinforcements. Journal of Composites Science, 5(8), 204. https://doi.org/10.3390/jcs5080204
- Yuan, X., Jiang, J., Wei, H., Yuan, C., Wang, M., Zhang, D., Liu, L., Huang, Y., Gao, G.-L., & Jiang, Z. (2021). PAI/MXene sizing-based dual functional coating for carbon fiber/PEEK composite. Composites Science and Technology. 201, 108496. https://doi.org/10.1016/j.compscitech.2020.108496
- Zhu, S., Shi, R., Qu, M., Zhou, J., Ye, C., Zhang, L., Cao, H., Ge, D., & Chen, Q. (2021). Simultaneously improved mechanical and electromagnetic interference shielding properties of carbon fiber fabrics/epoxy composites via interface engineering. Composites Science and Technology. 207, 108696. https://doi.org/10.1016/j.compscitech.2021.108696