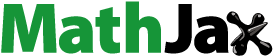
Abstract
This study examines how non-covalent and covalent surface modification methods affect the stability and thermo-physical characteristics of carbon nanotubes (CNTs) and graphene platelets (GNP) when distributed in solar thermal fluids. Surfactants are employed for examining non-covalent surface modification, while acid treatment is utilized for investigating covalent modification. Nanofluid samples are created with concentrations of 0.0625%, 0.125%, 0.25%, and 0.5% by weight in pure ethylene glycol (EG) and EG–water (80:20). Zeta potential analysis was utilized to study the stability of the nanofluids. The oxidized CNT–GNP nanofluids showed outstanding stability, with zeta potential values remaining almost constant for 60 d. Experiments were conducted on pure, surfactant-dispersed, and oxidized CNT–GNP nanofluids. The thermal conductivity of oxidized CNT–GNP nanofluids increased by 16% when 0.5 wt% was dispersed in the EG–water (80:20) sample. Nanofluids with CTAB and SDS showed a 14% and 13% enhancement in thermal conductivity, respectively. Formulas were created to forecast thermal conductivity and dynamic viscosity values.
1. Introduction
Hybrid nanofluids are a new blend of nanofluids with multiple nanoparticles dispersed in a base fluid. Researchers have shown increased interest in hybrid nanofluids for their improved thermophysical characteristics. Their enhanced heat transfer and thermal conductivity characteristics make them an ideal replacement for conventional fluids in numerous engineering applications. Hybrid nanofluids are gaining more popularity as an alternate heat transfer fluid in the area of solar thermal applications (Kazemian et al., Citation2022; Bao et al., Citation2023; Hu et al., Citation2021).
Carbon nanotubes (CNTs) have high thermal conductivity, making them a perfect candidate for nanofluids. Graphene platelets (GNPs) exhibit good mechanical strength and flexibility, thereby increasing nanofluid stability. Further, GNP’s two-dimensional structure enables it to form strong bonds with the base fluid molecules, making them easy to disperse. CNTs and GNPs complement each other, increasing the nanofluid’s overall heat transfer properties. GNPs have a two-dimensional structure, increasing the thermal conductivity perpendicular to the GNPs; combining CNT and GNP results in a more effective heat dispersion throughout the fluid.
Compared to other nanomaterials, CNTs and GNPs have specific advantages. Both exhibit exceptional strength because of their strong carbon bonds. Despite their strength, they are remarkably lightweight. They have high electrical and thermal conductivity, making them an excellent choice for applications involving heat transfer, energy storage devices, and automobile cooling systems. Furthermore, using chemical functionalization techniques, the properties of CNTs and GNPs can be tailored for specific applications.
The most commonly utilized base fluids are EG and water. EG has a high boiling point, making it a stable fluid at higher temperatures. In contrast, water has a high heat transfer coefficient and low viscosity, improving the fluid’s flow characteristics. The mixture of EG–water brings forth the benefits of water and EG. Mixing them in various proportions will help them be used in various engineering applications. Higher concentrations of EG in an EG–water mixture can be used in automotive cooling (Muruganandam & Mukesh Kumar, Citation2020; Devireddy et al., Citation2016). In contrast, lower EG concentrations can be used in solar collectors (Li et al., Citation2020; Xu et al., Citation2019), heat exchangers (Borode et al., Citation2019a; Askari et al., Citation2021), and cooling systems (Nazari et al., Citation2014). lists the current study’s base fluid properties.
Table 1. Basefluid properties.
CNTs often tend to agglomerate, making it difficult to uniformly disperse them in base fluids. To overcome this issue, researchers have used several surface modification techniques. Traditionally, researchers used non-covalent surface modification techniques like surfactant addition. These surfactants will reduce agglomeration by reducing the base fluid’s surface tension and stabilizes the nanofluids (Zhang et al., Citation2022; Ma et al., Citation2021; Zhai et al., Citation2019; Rehman et al., Citation2023). Several researchers used sodium dodecyl sulfate (SDS), polyvinylpyrrolidone (PVP), and cettabrimonium bromide (CTAB), etc. as surfactants.
When dispersing surfactants, the ratio of the surfactant to the CNT–GNP plays a crucial role in determining the stability of the nanofluids. Identifying the optimal ratio of surfactant to nanoparticles is critical to obtain a stable dispersion. Researchers (Al-Janabi et al., Citation2022; Kim et al., Citation2018) have observed that increasing the amount of surfactant in the nanofluid causes foaming and decreases its stability. This inconsistency can be mitigated by using covalent functionalization techniques.
Xing et al. (Citation2016) observed that MWCNTs with non-covalent surface modification, like adding CTAB surfactant, presented poor heat transfer coefficients, whereas covalent-modified MWCNTs displayed high heat transfer coefficients. Borode et al. (Citation2019b) studied the dispersion stability of CNT nanofluids when surfactants were used. They observed that increasing the surfactant concentration beyond a point did not improve the nanofluid stability. In addition, they observed that the surfactants adversely affected the dispersion stability above 60 °C.
Recently, researchers started using covalent surface modification techniques to improve the stability and dispersion of CNTs in liquids. Hussein Omar et al. (Citation2019) investigated various surface modification techniques on GNP nanofluids. They observed that covalent functionalized GNPs exhibited higher thermal conductivity when compared to non-covalent GNPs. Yellapu et al. (Citation2019) observed that covalent functionalized MWCNTs displayed excellent thermal and physical stability compared to pristine MWCNTs. One of the most popular techniques is treating CNTs with strong acids, forming a carboxylic acid group on the surface of CNTs. Alizadeh et al. (Citation2022) studied covalent and non-covalent functionalized MWCNTs and observed that the covalent functionalized MWCNTs exhibited improved heat transfer characteristics compared to the non-covalent MWCNTs. Almanassra et al. (Citation2020) conducted experiments to study the influence of PVP, SDS, and GA surfactants on aqueous CNT nanofluids. They observed that the PVP and SDS dispersed nanofluids were stable for 6 months, improving the thermal conductivity by 36%.
Lei et al. (Citation2022) investigate the influence of PVP, TMAH, and SDS surfactants on different thermophysical properties of Fe3O4 nanofluids. They observed that while SDS improved the thermal conductivity, PVP had a more significant impact on the dynamic viscosity. They also observed that adding TMAH gave the best stability to the nanofluids.
Although several researchers have observed thermal conductivity improvement using various surface modification techniques, there is a need to investigate and compare the impact of different surface modification techniques on ethylene glycol (EG)-based nanofluids. Therefore, this study investigates the influence of various surface modification techniques on EG–water-based CNT–GNP nanofluids’ stability, thermal conductivity, and dynamic viscosity.
2. Materials and methods
CNTs were purchased from M/s Cheap Tubes Inc. Grafton, VT, USA. These CNTs are 3–15 microns long and have a diameter between 30 and 50 nm. This study used analytical-grade chemicals.
2.1. Synthesis and characteristics of nanoparticles
CNTs are purified and oxidized using a three-step process. The initial step involves removing the amorphous carbon content from the CNTs. This purification is achieved by the chemical vapor deposition (CVD) process wherein the CNTs are heated at 575 °C for 45 min. Later, the CNTs are refluxed in four molar HCl for 4 h to remove the impurities left out by the CVD process. The next step involves covalent surface modification of the CNTs. Surface modification is done by oxidizing the CNTs for 3 h with 3:1 volume ratios of 4 molar H2SO4 and HNO3. To achieve a pH of 7, the CNTs are cleaned with water and then oven drying at 60 °C overnight.
The structure of CNTs was studied by a TEM (JEOL-2010, 200 kV). shows the procured CNTs with carbon black and metal impurities. These impurities cause the CNTs to agglomerate and get tangled. Also, the average length of the pristine CNTs was around 0.2 µm. shows the treated untangled CNTs with visible open ends without impurities. After treatment, the average length of the CNTs is reduced to 100 nm, which enables them to form stable dispersions in EG and water. The morphology of GNPs is also studied using a transmission electron microscope. shows the platelets with uniform size and a highly ordered lattice structure. It also shows the characteristic honeycomb pattern of the GNPs.
2.2. Preparation of nanofluids and stability
Two different compositions of base fluids are prepared for the current study. The base fluids composition is captured in . Oxidized CNTs with four different concentrations (0.0625%, 0.125%, 0.25%, and 0.5%) are first dispersed in the base fluid. An ultrasonicator was employed for 15 min to disperse the CNTs. Later, an equivalent amount of GNPs was added to each CNT/base fluid. For uniform dispersion of GNPs, a longer sonication time of 100 min was applied. Samples are also prepared using CTAB and SDS surfactants with a weight ratio of surfactants to CNT–GNP particles: (1:1), (2:1), and (3:1).
Table 2. Basefluid compositions.
The nanofluids are visually inspected for 2 months to determine their stability. Nanofluids are considered stable based on the duration taken without showing signs of sedimentation or agglomeration. The stability of the nanofluids was also analyzed using the zeta potential values. The zeta potential values for pristine, surfactant dispersed, and oxidized CNT–GNP fluids were measured on the 1st day, 30th day, and 60th day.
2.3. Thermophysical properties measurement
In fluids, heat transfer happens through convection, which is fluid movement because of agitation or turbulence. When measuring the fluid’s thermal conductivity, convection can cause the fluid to flow, resulting in inaccurate measurements. The transient plane source method mitigates these inaccuracies as the sensor is directly in liquid contact, reducing the risk of inaccuracies caused by convection. The current study used the C-Therm analyzer to measure thermal conductivity.
This study used the Brookfield DV-III rheometer to measure the samples’ dynamic viscosity. It calculates the viscosity based on the torque needed to rotate a spindle at constant velocity. As the device’s spindle rotates, the fluid resists the movement because of its viscosity, creating a torque proportional to the fluid’s viscosity. The device is connected to RheoCompass™software, allowing quick and precise measurement.
Thermal conductivity and viscosity measurements are taken for pristine, CTAB-dispersed (2:1), SDS-dispersed (2:1), and oxidized CNT–GNP nanofluids. The samples’ thermal conductivity and viscosity were measured at various temperatures. At a given temperature, the average value is calculated and reported to reduce the inaccuracies of the measurements.
3. Results and discussions
3.1. Nanofluids stability
Nanofluid stability is visibly inspected by observing the sedimentation of the nanoparticles in the base fluids for 2 months. Four samples of different mass concentrations (0.0625, 0.125, 0.25, and 0.5 wt%) were prepared by dispersing pristine CNT–GNP nanoparticles in EG–water base fluids. Samples are also prepared by dispersing CTAB and SDS surfactants to the nanofluids in three different surfactant to CNT–GNP ratios (1:1, 2:1, 3:1). Surfactants are added using the sonication process, which breaks the van der Waals forces of CNT particles and exposes the carbon atoms to the surfactant. CNT–GNP nanoparticles are oxidized with the help of acid treatment.
As part of stability observations, the samples with pristine CNT–GNP exhibited very low stability, as most of the nanoparticles formed sediments in the base fluids within the first 5 d. Nanofluids dispersed with CTAB and SDS in the ratio of (1:1) and (2:1) did not show any visible sediments indicating good stability. However, foam formation is observed for nanofluids with a CNT–GNP to surfactant ratio of 3:1. This suggests that increasing the surfactant concentration in the nanofluids will have a detrimental effect on stability. This foam formation at high concentrations of CTAB and SDS can be attributed to the formation of micelles. Micelles are spherical structures of self-assembled surfactant molecules. These micelles allow for the agglomeration of nanoparticles, thereby hindering the stability of the nanofluid. Nanofluids with oxidized CNT–GNP did not showcase any visible sediments even after 60 d.
Zeta potential measurements were taken for all the nanofluid samples at room temperature to validate the visual observations. It is often mentioned in the literature that fluids having absolute zeta potential values between 40 and 60 mV are highly stable (Sajid & Ali, Citation2018; Asadi et al., Citation2018; Van Trinh et al., Citation2018; Tiwari et al., Citation2021).
The zeta potential readings for pristine and oxidized CNT–GNP nanofluids are mentioned in and . In confirmation with the visual observations, the absolute zeta potential values of Pristine CNT–GNP nanofluid samples are less than 25 mV, indicating very poor stability. On the other hand, oxidized CNT–GNP nanofluids have absolute zeta potential values greater than 40 mV. The increased zeta potential values for oxidized CNT–GNPs are because of the functional groups on the CNTs’ surface. These functional groups increase the zeta potential value by creating an additional charge on the CNT’s surface. The zeta potential measurements for pristine and oxidized CNT–GNPs can be seen in and , respectively.
Figure 2. Zeta Potential of pure ethylene glycol dispersed with a) pristine 0.5% CNT–GNP on the first day and b) pristine CNT–GNP on the 5th day.

Figure 3. Zeta Potential of pure ethylene glycol dispersed with a) oxidized 0.5% CNT–GNP on the first day and b) oxidized CNT–GNP on the 60th day.

Table 3. Pristine CNT–GNP zeta potential values.
Table 4. Oxidized CNT–GNP zeta potential values.
It can be observed that both SDS and CTAB improved the stability of the nanofluids when added in the ratio of 1:1 and 2:1. However, for the 3:1 ratio, the zeta potential values are lower, which can be attributed to foam formation. The improvement in stability with surfactants is because they adsorb onto the nanoparticle’s surface and act as stabilizing agents. They create a repulsive force between the nanoparticles, preventing them from agglomerating. and show the zeta potential measurements of nanofluids dispersed with CTAB (2:1) and SDS (3:1). shows the zeta potential values of CTAB and SDS dispersed nanofluids in different weight ratios.
Figure 4. Zeta Potential of pure ethylene glycol dispersed with 0.5% CNT–GNP surface modified with CTAB with a ratio of (2:1) on a) the first day and b) the 60th day.

3.2. Thermal conductivity and dynamic viscosity
Heat transfer in CNTs occurs through the carbon atoms’ covalent bonds. When dispersed in the base fluid, they form a highly conductive pathway through which heat can flow. The nanofluid samples’ thermal conductivity was higher than the corresponding base fluids because of their enhanced thermal conductivity. It was also observed that higher nanoparticle concentration yielded higher thermal conductivity. However, samples dispersed in pure EG displayed lower thermal conductivity improvement because EG’s increased viscosity restricted the nanoparticles’ movement, resulting in lower thermal conductivity. illustrates the relation between thermal conductivity and temperature for EG: water (80:20) and pure EG nanofluids for pristine CNT–GNP nanoparticles. Thermal conductivity improved by 9%, 12%, 14%, and 16% for the 0.0625, 0.125, 0.25, and 0.5 wt% pristine CNT + GNP nanoparticles.
Figure 7. Variation of thermal conductivity for pristine CNT–GNP for a) EG–water (80:20) and b) Pure EG.

As indicated in , the thermal conductivity dropped with an increase in temperature. As temperature increases, the distance between the molecules increases, increasing the mean conduction path. This reduces the heat transfer capability of the fluid, thereby reducing its thermal conductivity. The thermal conductivity for pure EG nanofluids is lower when compared to EG-water (80:20) samples. This can be attributed to the increased viscosity of pure EG samples, restricting the free movement of nanoparticles in the base fluid.
The variation of dynamic viscosity with temperature for pure EG and EG–water (80:20) based nanofluids is illustrated in .
Figure 8. Dynamic viscosity variation of pristine CNT–GNP dispersed in a) EG–water (80:20) and b) Pure EG.

As evidenced in , nanoparticle concentration increases viscosity. It is also evident that an increase in temperature reduces viscosity. As temperature rises, the intermolecular attraction decreases, reducing the viscosity. However, viscosity variation is higher at lower temperatures; at higher temperatures, the viscosity variation between nanofluids and base fluids is negligible.
3.3. Effect of surface modification on thermal conductivity
The implemented surface modification technique significantly impacts the nanofluids’ thermal conductivity. These techniques improve thermal conductivity by improving the nanofluids’ stability. The influence of both non-covalent and covalent surface modification methods on thermal conductivity is investigated. Both CTAB and SDS have hydrophilic regions, making it easy to adsorb onto the CNT surface, uniformly disperse them, and increase the thermal conductivity.
Thermal conductivity can be further enhanced with covalent functionalization of CNT and GNP nanoparticles. Covalent functionalization mitigates foam formation and enhances thermal conductivity by forming carboxyl and hydroxyl groups on the CNT surface. This process altered the CNT surface structure, improving their reactivity and compatibility with polar solvents like water and EG. This improved compatibility leads to high stability, increasing the nanofluids’ thermal conductivity. The variation of thermal conductivity across all weight fractions for pristine, CTAB, SDS, and Oxidized nanofluids dispersed in EG–water (80:20) is depicted in .
Figure 9. Thermal conductivity variation across pristine, SDS, CTAB, and Oxidized CNT–GNP dispersed in EG–water (80:20) for a) 0.0625% b) 0.0125% c) 0.25% d) 0.5%.

For the nanofluid with EG–water (80:20), 0.5 wt% sample produced a 14% enhancement using CTAB and 13% using SDS. 0.5 wt% of oxidized CNT + GNP dispersed in EG–water (80:20) has produced a maximum thermal conductivity enhancement of 16%. displays the variation of thermal conductivity across all weight fractions for pristine, CTAB, SDS, and oxidized nanofluids dispersed in pure EG.
Figure 10. Thermal conductivity variation across pristine, SDS, CTAB, and oxidized CNT–GNP dispersed in pure EG for a) 0.0625%, b) 0.0125%, c) 0.25%, d) 0.5%.

The 0.5 wt% nanofluids dispersed in pure EG produced an 11% enhancement using CTAB and 9% using SDS, while the oxidized CNT + GNP dispersed in pure EG produced a maximum thermal conductivity enhancement of 14%. and show that the thermal conductivity is higher in oxidized nanofluids for all weight fractions. This higher thermal conductivity in oxidized nanoparticles can be attributed to the formation of stable bonds between the functional groups of the CNTs and the polar groups of EG and water.
3.4. Surfactants concentration impact on thermophysical properties
Surfactant concentration plays a crucial role in determining the nanofluids properties. Several studies (Gallego et al., Citation2020; Keklikcioglu Cakmak, Citation2020; Valan Arasu et al., Citation2019; Xia et al., Citation2014) have observed that increasing the surfactant concentration decreased the nanofluid’s thermal conductivity. At high concentrations, surfactants self-assemble to form micelles. These micelles encapsulate some nanoparticles within their core. These trapped nanoparticles become less effective in conducting heat, leading to a decrease in the overall thermal conductivity. shows thermal conductivity change against temperature for nanofluids dispersed with SDS and CTAB for surfactant to nanoparticle concentration of 3:1.
Figure 11. Thermal conductivity variation with temperature for nanofluids dispersed with surfactants for a) EG–water (80:20) and b EG 100%.

shows that the thermal conductivity of the nanofluids dispersed with surfactants had observed a significant drop in the thermal conductivity. For a surfactant-to-nanoparticle ratio of 3:1, the amount of surfactants is more than that of nanoparticles, hence decreasing the thermal conductivity.
depicts the dynamic viscosity of surfactant-dispersed nanofluids when the surfactant to nanoparticle concentration is 3:1.
Figure 12. Variation of viscosity with temperature for nanofluids dispersed with surfactants for a) EG–water (80:20) and b) Pure EG.

It is evident from that initially the viscosity is very high for the nanofluids dispersed with surfactants. However, as temperature increases, there is a steep decrease in viscosity. As mentioned already, higher concentrations of surfactants lead to micelle formation. At higher temperatures, the micelle structures start to dissociate, leading to a steep decrease in the viscosity.
3.5. Thermal conductivity and dynamic viscosity correlations
Several researchers have conducted experiments to understand the properties of nanofluids. However, some inconsistencies are observed in the results declared by the researchers. So, there is a need to identify a mathematical model that can predict nanofluids’ viscosity and thermal conductivity values. The presence of a mathematical model will reduce the repetition of experiments by researchers. It will also enable researchers to quickly estimate the data for previously unobserved data.
The regression model is developed using the Minitab software program. The input parameters are CNT–GNP nanoparticle concentration, EG vol%, and temperature. Thermal conductivity and dynamic viscosity are the predictors. The model with the lowest precision error is selected after multiple iterations. All variables are converted to log variables to reduce the prediction error and to fit a linear model. Later, the linear model is transformed into a non-linear model. The equation for thermal conductivity is represented by EquationEquation (1)(1)
(1) , and dynamic viscosity is represented by EquationEquation (2)
(2)
(2) .
(1)
(1)
(2)
(2)
Analysis is conducted to identify the relation between the predicted and experimental values. displays the plotting of the experimental values against the predicted values.
shows that the values predicted by the correlation formulas align with the experimental values. The forecasted values differ from the experimental values by a nominal range of ±5%.
4. Conclusions
The surface modification method used plays a critical role in the stability of CNT–GNP nanofluids. While pristine CNT–GNPs exhibited very low stability, CTAB and SDS-dispersed nanofluids exhibited good stability. Oxidized CNT–GNPs have the highest stability over 2 months based on zeta potential values.
Oxidized CNT–GNPs have produced the maximum thermal conductivity enhancement. This is because of the formation of stable hydroxyl and carboxyl bonds on the CNT surface. A maximum improvement of 16% in thermal conductivity is observed for the 0.5wt% sample dispersed in EG–water (80:20).
Viscosity of the nanofluids decreases with increase in temperature in the ranges of 50 °c–70 °C. In higher temperatures, the variation of viscosity is negligible. Similarly, the surface modification techniques have a negligible impact on the viscosity of the nanofluids.
Proposed correlation formulas for thermal conductivity and viscosity predicted the values with a maximum deviation of ±5%, indicating that the formulas can work for all concentrations of EG and water blends.
Author contributions
S. Bhanuteja: Data Collection, Experimentation, Manuscript Writing.
V. Srinivas: Conceptualization, Design, Experimentation, and Manuscript Writing.
K. Satyanarayana: Data Collection and Data Interpretation.
N. Darya Viktorovna: Methodology, Critical Feedback and Manuscript Review.
Ankita Joshi: Data Analysis and Data Visualization.
Acknowledgments
The authors gratefully acknowledge the support received from GITAM (Deemed to be University), and Osmania University, Hyderabad for conducting the tests.
Disclosure statement
No conflicts to disclose.
Data availability statement
The data that support the findings of this study are available from the corresponding author, V. Srinivas, upon reasonable request.
Additional information
Funding
References
- Alizadeh, H., Pourpasha, H., Zeinali Heris, S., & Estellé, P. (2022). Experimental investigation on thermal performance of covalently functionalized hydroxylated and non-covalently functionalized multi-walled carbon nanotubes/transformer oil nanofluid. Case Studies in Thermal Engineering, 31, 101713. https://doi.org/10.1016/j.csite.2021.101713
- Al-Janabi, A. S., Hussin, M., Abdullah, M. Z., & Ismail, M. A. (2022). Effect of CTAB surfactant on the stability and thermal conductivity of mono and hybrid systems of graphene and FMWCNT nanolubricant. Colloids and Surfaces A: Physicochemical and Engineering Aspects, 648, 129275. https://doi.org/10.1016/j.colsurfa.2022.129275
- Almanassra, I. W., Manasrah, A. D., Al-Mubaiyedh, U. A., Al-Ansari, T., Malaibari, Z. O., & Atieh, M. A. (2020). An experimental study on stability and thermal conductivity of water/CNTs nanofluids using different surfactants: A comparison study. Journal of Molecular Liquids, 304, 111025. https://doi.org/10.1016/j.molliq.2019.111025
- Asadi, A., Asadi, M., Rezaniakolaei, A., Rosendahl, L. A., Afrand, M., & Wongwises, S. (2018). Heat transfer efficiency of Al2O3-MWCNT/thermal oil hybrid nanofluid as a cooling fluid in thermal and energy management applications: An experimental and theoretical investigation. International Journal of Heat and Mass Transfer, 117, 474–486. https://doi.org/10.1016/j.ijheatmasstransfer.2017.10.036
- Askari, S., Ettefaghi, E., Rashidi, A., Seif, A., Rudd, J. A., Alonso, J. A., & Khodabakhshi, S. (2021). Ultra-stable nanofluid containing Functionalized-Carbon Dots for heat transfer enhancement in Water/Ethylene glycol systems: Experimental and DFT studies. Energy Reports, 7, 4222–4234. https://doi.org/10.1016/j.egyr.2021.07.001
- Bao, Y., Huang, A., Zheng, X., & Qin, G. (2023). Enhanced photothermal conversion performance of MWCNT/SiC hybrid aqueous nanofluids in direct absorption solar collectors. Journal of Molecular Liquids, 387, 122577. https://doi.org/10.1016/j.molliq.2023.122577
- Borode, A. O., Ahmed, N. A., & Olubambi, P. A. (2019a). Application of Carbon-based Nanofluids in Heat Exchangers: Current Trends. Journal of Physics: Conference Series, 1378(3), 032061. https://doi.org/10.1088/1742-6596/1378/3/032061
- Borode, A. O., Ahmed, N. A., & Olubambi, P. A. (2019b). Surfactant-aided dispersion of carbon nanomaterials in aqueous solution. Physics of Fluids, 31(7), 071301. https://doi.org/10.1063/1.5105380
- Devireddy, S., Mekala, C. S. R., & Veeredhi, V. R. (2016). Improving the cooling performance of automobile radiator with ethylene glycol water based TiO2 nanofluids. International Communications in Heat and Mass Transfer, 78, 121–126. https://doi.org/10.1016/j.icheatmasstransfer.2016.09.002
- Gallego, A., Cacua, K., Herrera, B., Cabaleiro, D., Piñeiro, M. M., & Lugo, L. (2020). Experimental evaluation of the effect in the stability and thermophysical properties of water-Al2O3 based nanofluids using SDBS as dispersant agent. Advanced Powder Technology, 31(2), 560–570. https://doi.org/10.1016/j.apt.2019.11.012
- Hu, G., Ning, X., Hussain, M., Sajjad, U., Sultan, M., Ali, H. M., Shah, T. R., & Ahmad, H. (2021). Potential evaluation of hybrid nanofluids for solar thermal energy harvesting: A review of recent advances. Sustainable Energy Technologies and Assessments, 48, 101651. https://doi.org/10.1016/j.seta.2021.101651
- Hussein Omar, A., Khairul, H., Saidur, R., Muhsan, S., Syed, S., & Alawi Omer, A. (2019). The influence of covalent and non-covalent functionalization of GNP based nanofluids on its thermophysical, rheological and suspension stability properties. RSC Advances, 9(66), 38576–38589. https://doi.org/10.1039/c9ra07811h
- Kazemian, A., Salari, A., Ma, T., & Lu, H. (2022). Application of hybrid nanofluids in a novel combined photovoltaic/thermal and solar collector system. Solar Energy, 239, 102–116. https://doi.org/10.1016/j.solener.2022.04.016
- Keklikcioglu Cakmak, N. (2020). The impact of surfactants on the stability and thermal conductivity of graphene oxide de-ionized water nanofluids. Journal of Thermal Analysis and Calorimetry, 139(3), 1895–1902. https://doi.org/10.1007/s10973-019-09096-6
- Kim, S., Tserengombo, B., Choi, S., Noh, J., Huh, S., Choi, B., Chung, H., Kim, J., & Jeong, H. (2018). Experimental investigation of dispersion characteristics and thermal conductivity of various surfactants on carbon based nanomaterial. International Communications in Heat and Mass Transfer, 91, 95–102. https://doi.org/10.1016/j.icheatmasstransfer.2017.12.011
- Lei, J., Luo, Z., Qing, S., Huang, X., & Li, F. (2022). Effect of surfactants on the stability, rheological properties, and thermal conductivity of Fe3O4 nanofluids. Powder Technology, 399, 117197. https://doi.org/10.1016/j.powtec.2022.117197
- Li, X., Zeng, G., & Lei, X. (2020). The stability, optical properties and solar-thermal conversion performance of SiC-MWCNTs hybrid nanofluids for the direct absorption solar collector (DASC) application. Solar Energy Materials and Solar Cells, 206, 110323. https://doi.org/10.1016/j.solmat.2019.110323
- Ma, M., Zhai, Y., Yao, P., Li, Y., & Wang, H. (2021). Effect of surfactant on the rheological behavior and thermophysical properties of hybrid nanofluids. Powder Technology, 379, 373–383. https://doi.org/10.1016/j.powtec.2020.10.089
- Muruganandam, M., & Mukesh Kumar, P. C. (2020). Experimental analysis on internal combustion engine using MWCNT/water nanofluid as a coolant. Materials Today: Proceedings, 21, 248–252. https://doi.org/10.1016/j.matpr.2019.05.411
- Nazari, M., Karami, M., & Ashouri, M. (2014). Comparing the thermal performance of water, Ethylene Glycol, Alumina and CNT nanofluids in CPU cooling: Experimental study. Experimental Thermal and Fluid Science, 57, 371–377. https://doi.org/10.1016/j.expthermflusci.2014.06.003
- Rehman, A., Yaqub, S., Ali, M., Nazir, H., Shahzad, N., Shakir, S., Liaquat, R., & Said, Z. (2023). Effect of surfactants on the stability and thermophysical properties of Al2O3 + TiO2 hybrid nanofluids. Journal of Molecular Liquids, 391, 123350. https://doi.org/10.1016/j.molliq.2023.123350
- Sajid, M. U., & Ali, H. M. (2018). Thermal conductivity of hybrid nanofluids: A critical review. International Journal of Heat and Mass Transfer, 126, 211–234. https://doi.org/10.1016/j.ijheatmasstransfer.2018.05.021
- Tiwari, A. K., Pandya, N. S., Said, Z., Öztop, H. F., & Abu-Hamdeh, N. (2021). 4S consideration (synthesis, sonication, surfactant, stability) for the thermal conductivity of CeO2 with MWCNT and water based hybrid nanofluid: An experimental assessment. Colloids and Surfaces A: Physicochemical and Engineering Aspects, 610, 125918. https://doi.org/10.1016/j.colsurfa.2020.125918
- Valan Arasu, A., Dhinesh Kumar, D., & Idrish Khan, A. (2019). Experimental investigation of thermal conductivity and stability of TiO2-Ag/water nanocompositefluid with SDBS and SDS surfactants. Thermochimica Acta, 678, 178308. https://doi.org/10.1016/j.tca.2019.178308
- Van Trinh, P., Ngoc Anh, N., Tuan Hong, N., Ngoc Hong, P., Ngoc Minh, P., & Hung Thang, B. (2018). Experimental study on the thermal conductivity of ethylene glycol-based nanofluid containing Gr-CNT hybrid material. Journal of Molecular Liquids, 269, 344–353.https://doi.org/10.1016/j.molliq.2018.08.071
- Xia, G., Jiang, H., Liu, R., & Zhai, Y. (2014). Effects of surfactant on the stability and thermal conductivity of Al2O3/de-ionized water nanofluids. International Journal of Thermal Sciences, 84, 118–124. https://doi.org/10.1016/j.ijthermalsci.2014.05.004
- Xing, M., Yu, J., & Wang, R. (2016). Effects of surface modification on the pool boiling heat transfer of MWNTs/water nanofluids. International Journal of Heat and Mass Transfer, 103, 914–919. https://doi.org/10.1016/j.ijheatmasstransfer.2016.07.053
- Xu, X., Xu, C., Liu, J., Fang, X., & Zhang, Z. (2019). A direct absorption solar collector based on a water-ethylene glycol based nanofluid with anti-freeze property and excellent dispersion stability. Renewable Energy, 133, 760–769. https://doi.org/10.1016/j.renene.2018.10.073
- Yellapu, G., Chachin Vishal, C. V., Pramod Kandoth, M., Saha, P., Bojja, R. R., Gandham, S., & Kanaparthi, R. (2019). Functionalized multi-walled carbon nanotubes based Newtonian Nano fluids for medium temperature heat transfer applications. Thermal Science and Engineering Progress, 12, 13–23. https://doi.org/10.1016/j.tsep.2019.04.014
- Zhai, Y., Li, L., Wang, J., & Li, Z. (2019). Evaluation of surfactant on stability and thermal performance of Al2O3-ethylene glycol (EG) nanofluids. Powder Technology, 343, 215–224. https://doi.org/10.1016/j.powtec.2018.11.051
- Zhang, H., Qing, S., Xu, J., Zhang, X., & Zhang, A. (2022). Stability and thermal conductivity of TiO2/water nanofluids: A comparison of the effects of surfactants and surface modification. Colloids and Surfaces A: Physicochemical and Engineering Aspects, 641, 128492. https://doi.org/10.1016/j.colsurfa.2022.128492