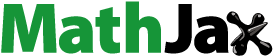
Abstract
Using high-filled cut-and-cover tunnels (HFCCTs) can provide a practical and ideal solution for the possibility of using highly demanded lands with plateaus terrain. The huge amount of HFCCT backfill soil produces ultrahigh vertical earth pressure (VEP). Therefore it is necessary to use load reduction methods and mechanisms to reduce the VEP on the HFCCT, which will reduce the risks and increase safety by reducing the tunnel designing loads. This experimental study describes methods of VEP reduction on the HFCCTs using expanded polystyrene (EPS) geofoam as a compressible material in two different forms without and with the presence of geogrid on top of the EPS. Several important factors, including the effect of geogrid presence on top of the EPS, the EPS form, the EPS thickness, and the distance between the bottom of the EPS and the top of the HFCCT discussed and studied. It determined from the study results that the mentioned factors have significant effects on the VEP reduction on the HFCCTs through their roles in the VEP reduction mechanisms. This study adopted two VEP reduction mechanisms; relative vertical displacements of the HFCCT backfill soil prisms and soil arching. The mentioned two mechanisms caused a significant reduction in the VEP on the top of the HFCCT, where the best result was a 76.0% reduction in the VEP on the HFCCT.
Reviewing Editor:
1. Introduction
The HFCCTs provide a reasonable and practical solution for the possibility of reclaiming valuable lands with valleys and hilly or mountainous terrain around the world. The basic property of the HFCCTs is their very high or huge backfill soil. However, the ultrahigh high earth pressure induced on the top or side of the HFCCT can cause serious structural damages and problems and safety related concerns. Studies focusing on the pressure reduction effect in the backfill soil above pipes and culverts took place since the early years of the 20th century; but, a few or very limited studies were focusing on HFCCTs related load and load reduction issues.
Marston initiated the idea and concept of rigid pipes installation in a trench buried under backfill soil. Earth pressure on buried rigid pipes is usually estimated using Marston’s theory (Marston and Anderson, Citation1913; Marston, Citation1930).
In 1922 Marston confirmed that the major factor which affects the earth pressure on underground rigid pipes is depending on the relative settlement between the soil column (interior prism) above the rigid pipe and the adjacent soil columns (exterior prisms) that control the magnitude and direction of friction, which affecting the earth pressure on the rigid pipe. A compressible material, like baled straw, leaves, woodchips or sawdust, and expanded polystyrene (EPS) geofoam, can be placed above rigid pipes to reduce the earth pressure on the rigid pipes and produce positive soil arching (Gu et al., Citation2005; Kang et al., Citation2007; Li et al., Citation2019; McAffee and Valsangkar, Citation2008; McGuigan and Valsangkar, Citation2010; Sladen and Oswell, Citation1988; Spangler, Citation1958; Taylor, Citation1973; Vaslestad et al., Citation1993).
In northwestern China, the HFCCTs are prevalent because the construction of HFCCTs allows the reclaiming of valuable lands. Because of the unique plateau topography of this region of China, the required quantities of backfill soil for HFCCTs are massive, and the backfill must be high enough to increase the usable land area. At present, the main challenge of HFCCTs construction is high earth or ground pressure on the HFCCTs lining structure and related safety concerns (Li et al., Citation2020).
A study was conducted to discuss laboratory experimental tests which were made to examine ways of earth pressure reduction methods by inserting expanded polystyrene (EPS) geofoam into the backfill soil and modifications to the structure of HFCCTs. The results of the experimental tests are in good agreement with the results of the numerical analysis. Numerical analysis was used to determine the optimal thicknesses of EPS to reduce the earth pressure on HFCCT with different backfill heights. Modifying the sectional shape of HFCCT can change the internal forces and make the concrete structure of HFCCT support more compressive loads instead of failing due to bending moments. The results of the study show that the dual effects of load reduction with EPS and sectional modifications of HFCCT can reduce the required thickness of the HFCCT structure, increase the allowable backfill height, and improve the HFCCT safety (Li et al., Citation2020).
A research was conducted to describe three load reduction scenarios: using EPS geofoam on the top of the HFCCT; a combination of EPS geofoam on top of the HFCCT and geogrid above the EPS geofoam; and a combination of EPS geofoam on the top of the HFCCT, geogrid above the EPS geofoam, and concrete wedges under the side edges of the geogrid. PFC2D computer software is used to analyze the suggested load reduction scenarios. Significant factors including: density, thickness, width, and the location of the EPS geofoam over the HFCCT, in addition to the number of layers and the tensile strength of the geogrid were studied. The analysis results were based on the changes in the VEP, the backfill soil relative vertical displacement, the contact force among the particles of backfill soil, and the geogrid relative vertical displacement. The research results determined that the factors have significant effects on the load reduction mechanisms. To optimize the earth pressure reduction on the top of HFCCTs, the influential factors’ optimum values were derived (Li, Yao, et al., Citation2020).
Better methods to reduce loads on the HFCCTs, which will reduce lining structure and design costs and increase safety, need a good understanding of the load or earth pressure reduction mechanisms. The earth pressure on top of HFCCTs can be reduced using relatively low compacted (RLC) soil, but using the RLC soil layer on the top of HFCCTs makes the load transfer mechanism more complex. The prior studies have either focused on the micromechanical properties of backfill soils or ignored their special properties. Therefore, if the micromechanical properties of the backfill soil’s can be correctly considered, then the load transfer mechanisms can be better understood. It should be considered to backfill the HFCCTs with soils with different relative compaction (R) percentages, such as R = 90% for HFCCT main Backfill soil and R = 80% for RLC layer above the HFCCT (Li et al., Citation2019).
The discrete element method (DEM) was employed to examine and inspect the changes in vertical earth pressure (VEP) on HFCCTs proportional to the thickness and spread distance of the RLC soil layer, the valley width to the width of the HFCCT ratio (the B/D ratio), and the slope angle. To characterize these factors, parametric DEM studies were conducted. The results of the DEM study showed that an adequate thickness and spread distance of the RLC soil layer could optimize the effect of soil arching and reduce VEP on top of HFCCTs. Also, the results showed that the slope angle and B/D ratio are related to the redirect of the VEP (Li et al., Citation2019).
Tire-derived aggregate (TDA) was successfully applied to reduce the earth pressure of backfill soil on a railway CCT. Numerical analyses were conducted to check and examine the effect of different assumptions about the base model with the suggested TDA applying formations around the CCT. Numerical analysis results stated that up to 60% reductions in the tunnel lining structure flexural moment can be achieved. For the studied case, the TDA elastic property has a small effect on CCT lining pressures in despite of it is fundamental for the CCT backfill settlement estimates (Rodríguez et al., Citation2018).
A numerical study conducted to examine two load reduction methods using a combination of TDA and geogrid. Abaqus 2019 software was employed to analyze the LEP reduction progress and mechanism. Several factors, including the geogrid existence, the form of the TDA, the thickness of the TDA, and the distance between the bottom of the TDA and top of the HFCCT and were studied. It was determined that the factors are having significant effects on the LEP reduction on the HFCCT through the earth pressure reduction mechanisms, where the average LEP on the top of the HFCCT model reduced from 303 kPa to 125 kPa (Arkawazi and Hajiazizi, Citation2023).
Currently, the existing methods of the VEP estimation may not suit the construction of the HFCCTs. The ability to estimate the earth pressure on the HFCCTs is important. Basically, the VEP on the HFCCTs can be estimated using soil column pressure equation. A numerical investigation conducted to measure the effect of using EPS geofoam and TDA in different formations on the relative vertical displacements in the HFCCT backfill soil and VEP reduction on the HFCCTs. The results of the numerical investigation showed a significant VEP reduction on the HFCCTs, especially by using the TDA in different formations. Then, a comparison is made between the estimated and calculated VEP values on the top of the HFCCT study model with the base conditions using Abaqus CAE 2019 software, the soil column pressure equation, and Li et al. (Citation2019) modified equation. The estimated and calculated VEP values on the top of the HFCCT study model with the base conditions showed that the estimated value using Abaqus CAE 2019 is 0.156% higher than the calculated value using the soil column pressure equation, which is a low percentage of difference. While the calculated value using Li et al.’s (Citation2019) modified equation is 27.942% lower than the calculated value using the soil column pressure equation, which is a high percentage of difference (Arkawazi and Hajiazizi, Citation2022).
This study presents an experimental investigation of the effect of using EPS as a compressible material and the associated effect of using EPS as a compressible material and geogrid through four scenarios of VEP reduction on HFCCTs. The study includes using EPS and geogrid in two different formations:
In a horizontal formation (without and with the presence of geogrid above the EPS),
In a combined horizontal and arch formation (without and with the presence of geogrid above the EPS).
The EPS in the four scenarios is located above the HFCCT concrete lining structure with six thicknesses and three distances between the top of the HFCCT and bottom of the EPS.
Some factors, such as the geogrid existence effect, the EPS formation, the EPS thickness, and the distance between the top of the HFCCT and the bottom of the EPS, will be checked and examined. The study results will focus on changes in VEP, relative vertical displacement in the backfill soil prisms, and the geogrid existence effect on the top of the EPS.
2. The HFCCT study model
For purposes of this study, a prototype HFCCT model was selected. The HFCCT study model consists of a 50 m depth valley and two sides slope with 70° angles, a 4-lane road CCT (the CCT height is 7.7 m) is located at the base of a valley. The selected study model can be considered quite challenging in terms of the very high earth pressure applied on the HFCCT produces from the huge backfill soil amount. The HFCCT study model parameters were selected to reflect the natural difficult conditions of valleys and the hilly terrain.
In general, HFCCT has a 30-50 m backfill soil height, such a high soil column produces very high earth pressure on the lining structure of the CCT, and the structure is susceptible to crack, causing damages to the tunnel structure and difficulty in its regular function (Zhuo et al., Citation2020).
shows the cross-section of the HFCCT study model with all the required details and dimensions to conduct the current study.
3. Material parameters
3.1. HFCCT backfill soil physical and mechanical properties
The required physical and mechanical properties of the backfill soil to conduct this study were determined through conducting the relevant laboratory tests. The internal friction angle, φ, the cohesion, c, and Young’s modulus values were determined by performing triaxial CD compression tests. The soil classification according Unified Soil Classification System (USCS) is clayey sand (CS). The soil samples were taken from the west of Iraq, which is an area with plateaus terrain. The backfill soil tests results are shown in .
Table 1. The physical and mechanical properties of the HFCCT backfill soil.
3.2. EPS geofoam physical and mechanical properties
Expanded polystyrene (EPS) geofoam is a rigid cellular plastic foam that has been widely used in geotechnical applications, including modification and improving of mechanical properties of soil (Bazzazian Bonab et al., Citation2021; Hassan et al., Citation2021; Jili et al., Citation2022; Khan and Meguid, Citation2018; Nawghare and Mandal, Citation2020; Ram Rathan Lal and Nawkhare, Citation2016), stabilization of slopes (Jutkofsky et al., Citation2000), rapid construction of embankments over compressible soils (Farnsworth et al., Citation2008; Khan and Dasaka, Citation2020a; Shafikhani et al., Citation2017), wave barrier (Khan and Dasaka, Citation2020b), lateral loads reduction (static and dynamic) on retaining walls and bridge abutments (Bathurst et al., Citation2007; Gade and Dasaka, Citation2022; Hatami and Witthoeft, Citation2008; Horvath, Citation1998; Citation2004; Khan and Meguid, Citation2021; Sravanam and Mouli, Citation2022; Xie et al., Citation2020; Zarnani and Bathurst, Citation2007; Citation2008), as a dynamic loads damper or barrier (Ossa and Romo, Citation2011) and as a sub-base filling material (see ) (Dugkov, Citation1998; Dugkov and Scarpas, Citation1998; Huang and Negussey, Citation2011; Stark et al., Citation2004). Numerical solutions were used to study EPS geofoam composite soil with its physical and engineering properties (Gao, Chen et al., Citation2011; Gao, Liu et al., Citation2011). The EPS is widely used in geotechnical applications because of its unique properties, such as being lightweight (low density), having different mechanical behavior, and being low in permeability. Several studies have shown that the compressive strength of EPS Geofoam is mainly dependent on material density, confining stress, and strain rate (Chun et al., Citation2004; Hazarika, Citation2006; Leo et al., Citation2008; Ossa and Romo, Citation2009; Wong and Leo, Citation2006).
Figure 2. Using EPS geofoam in railway embankment construction (Unipod, Citation2021).

Figure 3. Using EPS geofoam in road construction (Unipod, Citation2021).

Figure 4. Using EPS geofoam in a culvert fill over (Unipod, Citation2021).

For the past forty years, EPS has been successfully used as construction material in civil engineering projects, especially in the field of geotechnical engineering, due to its wide variety and range of applications, such as in embankments as lightweight filling material and in retaining walls as a compressible inclusion (Padade and Mandal, Citation2012). EPS is an attractive area in the geotechnical field of civil engineering, where its service life and durability is similar to other construction materials. Furthermore, it can use in backfilling highways’ sloped sides and vertical embankments. Also, as a compressible material below slabs or beams at a foundation, and above pipelines, culverts, and tunnels (Beju and Mandal, Citation2017b); Abdollahi et al., Citation2019; Meguid and Hussein, Citation2017; Meguid et al., Citation2017; Beju and Mandal, Citation2017a; Meguid and Ahmed, Citation2020).
For research purposes, four different densities of EPS geofoam are selected and purchased from EPS geofoam local suppliers in Mumbai, India. shows the EPS geofoam line sketch, and shows the photograph of the triaxial test EPS geofoam specimen used in the experimental study tests. The mechanical properties of the EPS geofoam are given and illustrated in (Padade and Mandal, Citation2012).
Figure 5. Triaxial test specimen of EPS geofoam (a) line sketch and (b) photograph (Padade and Mandal, Citation2012).

Table 2. Mechanical properties of EPS geofoam (Padade and Mandal, Citation2012).
A standard triaxial loading frame with a triaxial cell to fit a sample with 75 mm diameter and 150 mm height is used to conduct the experimental tests (see ). The Unconsolidated Un-drained triaxial experimental tests were conducted with a constant strain rate of 1.2 mm/min and were performed according to IS 2720 (Part 11):1993. A load cell and Linear Variable Differential Transducer were used to measure the deviator load and vertical displacement. All the tests were conducted with up to a maximum axial strain of 15% (Padade and Mandal, Citation2012).
Figure 6. Triaxial test on EPS geofoam (a) Placement of EPS Geofoam test specimen and (b) EPS geofoam specimen during the test (Padade and Mandal, Citation2012).

In the testing of the EPS geofoam specimens under triaxial loading conditions, no particular surface failure was observed. It was noticed that the specimens were getting marginal and compressed or small sideways bulging with the increase in the deviator load. In all the EPS geofoam confining pressures and densities, a similar pattern was observed. The EPS geofoam test specimen deformation with different densities is shown in (Padade and Mandal, Citation2012).
Figure 7. Deformation of the EPS geofoam test specimen under different confining pressures for various unit weights (a) 0.15 kN/m3, (b) 0.20 kN/m3, (c) 0.22 kN/m3, and (d) 0.30 kN/m3 (Padade and Mandal, Citation2012).

Deviator load was noted or recognized for each increase of axial deformation of EPS geofoam specimen. Deviator stress-strain relationships of EPS geofoam with different unit weights under different confining pressures are shown in . For all the tested unit weights of EPS geofoam, the stress-strain relationship was linear up to the axial strain value of around 2%; later, it was observed that there was no significant change in the deviator stress value with respect to the increase in axial strain value. As the unit weight of the EPS geofoam is increased, the deviator load is increased for all the confining pressures. For all the unit weights and confining pressures, the principal stress difference was almost equal (Padade and Mandal, Citation2012).
Figure 8. Stress-strain relationship of EPS geofoam under triaxial loading for different unit weights (a) 0.15 kN/m3, (b) 0.20 kN/m3, (c) 0.22 kN/m3, and (d) 0.30 kN/m3 (Padade and Mandal, Citation2012).

By constructing Mohr’s circles, the EPS geofoam strength parameters of different unit weights were calculated (see ). The EPS geofoam cohesion value increased with the increasing in the unit weight, and there was slight increase in the internal friction angle. The EPS geofoam strength parameters obtained from the triaxial tests are shown in . From the strength parameters, it was noticeable that the cohesion value has a considerable effect on the EPS geofoam strength. shows the relation of cohesion values with respect to the EPS geofoam corresponding unit weight. A regression analysis is conducted for different EPS geofoam unit weights and the best fitted to a curve expressed as EquationEquation 1(1)
(1) (Padade and Mandal, Citation2012).
(1)
(1)
Figure 9. Mohr’s circle construction for different unit weights of EPS geofoam (a) 0.15 kN/m3, (b) 0.20 kN/m3, (c) 0.22 kN/m3, and (d) 0.30 kN/m3 (Padade and Mandal, Citation2012).

Figure 10. Correlation between cohesion and unit weight of EPS geofoam (Padade and Mandal, Citation2012).

Table 3. Shear strength parameters of EPS geofoam of different unit weights (Padade and Mandal, Citation2012).
Where:
C: is the cohesion (kPa).
γg: is the unit weight of EPS geofoam (kN/m3).
The EPS geofoam Poisson’s ratio (υ) value is calculated using EquationEquation 2(2)
(2) (Padade and Mandal, Citation2012). The Poisson’s ratio (υ) value and other EPS geofoam properties are illustrated in .
(2)
(2)
Table 4. EPS geofoam material properties (Padade and Mandal, Citation2012).
Where:
ρ: Density of EPS geofoam (kg/m3).
For the purposes of this study and to achieve highest relative settlement between the HFCCT backfill soil interior and exterior prisms, the EPS geofoam with a 15 Kg/m3 density is selected. The mechanical and physical properties of the selected EPS geofoam are given in .
3.3. Geogrid physical and mechanical properties
Geogrids usually are made of polymeric materials such as high-density polyethylene, polyester, and polypropylene. Geogrids manufacturing includes a different process (extruded and punched-drawn, welding, or knitting) (Koerner, Citation2005). The geogrids manufactured by the extruded and punched-drawn have rigid joints at nodes and are unitized, and the angle between two adjacent ribs does not change during loading due to much larger thickness at nodes than ribs. The geogrids were manufactured with apertures in a square or rectangular shape and were used to carry tensile forces in one or two directions along the ribs. The one-directional tensile strength geogrid is known as uniaxial geogrid and is used for slopes and walls (Han and Leshchinsky, Citation2010). The two-directional tensile strengths geogrid is named bidirectional or biaxial geogrid and is used for pile-supported embankments, roads, and foundations. Geogrid’s uses have been increasing over the past 30 years, and it is expected to keep expanding and rising. About ten years ago, the geogrid with a triangular aperture was introduced into the market. The triangular aperture geogrid is manufactured with three equilateral directions oriented ribs and is expected to have a more stable grid structure and provide more uniform resistance to tensile forces in all directions, and is expected the geogrid with triangular apertures to be used in similar applications as bidirectional (biaxial) geogrid especially when the loading is not only in two directions (Dong et al., Citation2010). shows the rectangular and triangular apertures geogrid. The uses of bidirectional geogrids for foundation reinforcement, subgrade improvement, pile-supported embankments, soil reinforcement, buried flexible pipes protection and base and ballast reinforcement studied by many researchers (Abdullah and Edil, Citation2007; Adams and Collin, Citation1997; Alotaibi et al., Citation2021; Brown et al., Citation2007; Fattah, Zbar and Al-Kalali, Citation2023; Han and Akins, Citation2002; Helstrom et al., Citation2006; Huang and Han, Citation2009; Kinney et al., Citation1998; Tang et al., Citation2008). includes some of the studies conducted using different types of geogrids with their properties, and shows the typical geogrids with rectangular and triangular apertures. For the purposes of this study, the required geogrid physical and mechanical properties were selected and summarized in .
Figure 11. Rectangular and triangular apertures geogrid products (extruded and punched-drawn) (a) Rectangular apertures geogrid, (b) Triangular apertures geogrid (Dong et al., Citation2010).

Figure 12. Various types of geogrid with different manufacturing materials (Al-Barqawi et al., Citation2021).

Table 5. Some studies conducted using different types of geogrids with their properties.
Table 6. Geogrid required physical and mechanical properties required for the numerical analysis (Tharani et al., Citation2019).
4. Physical experimental model
For the physical experimental model tests, a rigid steel tank with the dimensions of 200 cm × 100 cm × 100 cm (length × width × height) was made and used. The front and rear of the tank are made of thick glass to observe the soil displacement during backfilling. The glass surfaces of the front and rear faces of the experimental model are smooth, and the inside of the front and back glass faces are painted with lubricant oil to eliminate or minimize the friction between the backfill soil and the glass surface. In the tank, a high filled cut and cover tunnel model with an arched cross-sectional shape was installed at the center of the bottom. The high filled cut and cover tunnel (HFCCT) model is manufactured with a length of 100 cm, a height of 15.4 cm, and a width of 30.8 cm. The slope angle of the two side slopes of the HFCCT experimental model is 70°. The two slopes are assumed to be rigid so steel sheets were used to form the slopes, and the side slopes surfaces were made to be rough to simulate the friction effect between the side slopes and the HFCCT backfill soil (see ).
In the experimental tests, to reflect the real conditions, the tests were performed according to the model scale, and corresponding parameters between the experimental model and the prototype were calculated as a similarity scale for each parameter. Totally as part of this research, 73 experimental tests were conducted.
4.1. Scale analysis for the experimental model and tests
To reflect real conditions in the experimental tests and make tests results applicable to the prototype, a correlation of the scale of the experimental model with that of the prototype is required. In this study, similar prototype materials were used to establish the experimental model, therefore the density similarity ratio (SR𝛾) for the testing materials is 1. The model scale ratio for the experimental model is 1/50, which was determined based on the research goals and objectives and appropriate test tools and devices. Accordingly, the experimental model similarity ratio (SRm) is 50. The corresponding parameters between the experimental model and the prototype are calculated and listed in . Where: SRσ is similarity scale of stress, SRɛ is similarity ratio of strain SRE is similarity scale of elastic modulus; SRυ is similarity scale of Poisson’s ratio; SRu is similarity scale of displacement (Wood, Citation2017).
Table 7. General scale ratios of experimental model and tests (Wood, Citation2017).
4.2. Experimental tests procedures and measurements
To simulate the actual HFCCT backfilling process, the following steps were taken for each experimental test:
Placing the HFCCT at the center of the bottom, and then installing two earth pressure measurement cells between the side slopes and the sides of the HFCCT [see ].
Fill and compact the first backfill soil layer with a height that reaches the top of the HFCCT (15.4 cm) [see ].
Install five earth pressure measurement cells after the first backfill soil layer is filled and compacted [see ].
Complete the second backfill layer works according to the required distance between the top of the HFCCT and the bottom of the EPS for the specific conducted experimental test.
Install the EPS with the specific form as required for each experimental test. This process will be conducted without and with the presence of geogrid above the EPS for each of the EPS formations [see as an example].
Mark the line for the HFCCT backfill soil vertical displacement measurements.
Repeat the backfilling process of filling and compacting until reaching the required HFCCT backfill height.
For each experimental test, record the vertical earth pressure (VEP) and vertical displacement of the backfill soil after finishing all the backfilling works.
Figure 14. The setup of experimental tests: (a) Placing the HFCCT at the center of the bottom, and then installing two earth pressure measurement cells between the side slopes and the sides of the HFCCT, (b) Fill and compact the first backfill soil layer with a height that reaches the top of the HFCCT (15.4 cm), (c) Install five earth pressure measurement cells after the first backfill soil layer is filled and compacted, and (d) Install the EPS and the geogrid with the specific formation as required for each experimental test.

The experimental model tests used DM-YB1820 Dynamic and Static Strain Test System (20 channel, 10 Hz model data logger) (see and ) and seven earth pressure measurement cells, type miniature pressure cells with a 28 mm diameter and 10 mm thickness (see ), to measure vertical earth pressure values around the HFCCT. The measuring capacity of the earth pressure measurement cells is 0.1 Mpa with 0.1% sensitivity. Plastic strips were used to mark lines in the HFCCT backfill soil to observe any vertical displacement in the backfill soil. The deformation of the reinforced concrete structure of the HFCCT was small, so the deformation of the HFCCT and its effect on the backfill soil vertical displacement were ignored.
Figure 16. Connection diagram of DM-YB1820 Dynamic and Static Strain Test System (20 channel, 10 Hz model data logger) and Sensors (Xian Yima Optoelec Limited Company, Citation2022).

5. Experimental tests
As part of this research, the following experimental tests were conducted:
5.1. Experimental test for the HFCCT physical experimental model with the base conditions (no-load reduction method is used)
For the evaluation purposes of the HFCCT experimental model with the base conditions, a test was conducted to measure the VEP on the top of the HFCCT experimental model in addition to measuring the vertical displacement in the backfill soil on the HFCCT experimental model with 90 mm (10 mm the thickness of the miniature pressure cell plus the highest thickness of EPS or TDA which is 60 mm plus 20 mm which is the highest distance between the top of the HFCCT and the bottom of the EPS or TDA) (see ). The measured VEP experimental value on the top of the HFCCT full-scale model for the base conditions (no-load reduction method is used) was 716.6 kPa.
5.2. Experimental tests for the HFCCT physical experimental model with load reduction method using EPS geofoam in a horizontal form
18 tests were conducted using EPS geofoam in a horizontal form on top of the HFCCT experimental model. In these tests, 6 EPS thicknesses of 10 mm, 20 mm, 30 mm, 40 mm, 50, and 60 mm were used with three distances of 5 mm, 10 mm, and 20 mm between the top of the HFCCT experimental model and the bottom of the EPS. In each test, measurements of VEP on the top of the HFCCT experimental model and in other parts of the backfill soil, in addition to measuring the vertical displacement in the backfill soil, were taken after finishing the backfill process (see ).
Figure 19. Experimental test for using EPS geofoam in a horizontal form as a method of VEP reduction on the HFCCT research experimental model.

Within the results of the experimental test results converted for the full-scale HFCCT research model, and present the relationships of the VEP and the percentage of reduction in the VEP on the HFCCT research model with the EPS thickness (EPS is in a horizontal form) and the distance between the top of the HFCCT and the bottom of the EPS. For all the EPS thicknesses, as the thickness of the EPS increases, the vertical earth pressure on the HFCCT is reduced. The best result of vertical earth pressure reduction on the HFCCT was obtained when the EPS thickness is 3 m and the distance between the top of the HFCCT and the bottom of the EPS was 0.25 m, where the VEP on the HFCCT reduced from 716.6 kPa to 383.6 kPa (46.4% reduction in the VEP on the HFCCT) (see and ).
Figure 20. The relationship of the VEP on the HFCCT research model with the EPS thickness (in a horizontal form) and the distance between the top of the HFCCT and the bottom of the EPS.

Figure 21. The relationship of the percentage of reduction in the VEP on the HFCCT research model with the EPS thickness (in a horizontal form) and the distance between the top of the HFCCT and the bottom of the EPS.

The EPS geofoam inclusion in a horizontal form leads the soil prism (interior prism) within the HFCCT structure width to settle more than the surrounding soil prisms (exterior prisms) due to deformation of the EPS (see ), the soil prism (interior prism) within the HFCCT structure width deform as a reverse arch-shape. Meanwhile, the reduced VEP on the HFCCT structure is equal to the shear force on the soil in the interior prism.
Figure 22. Measured vertical displacement of the backfill soil on the HFCCT research experimental model at a 90 mm backfill height in the experimental tests using EPS in a horizontal form.

The results of the load reduction method using EPS geofoam in a horizontal form indicate that the increase in distance between the EPS bottom and the top of the HFCCT does not enhance the soil arching effect but instead increases the VEP on the HFCCT. Therefore, the optimum distance can be considered the shortest distance between the EPS bottom and the top of the HFCCT.
5.3. Experimental tests for the HFCCT physical experimental model with load reduction method using EPS geofoam in a combined horizontal and arch form
18 tests were conducted using EPS geofoam in a combined horizontal and arch form on top of the HFCCT experimental model. In these tests, 6 EPS thicknesses of 10 mm, 20 mm, 30 mm, 40 mm, 50, and 60 mm were used with three distances of 5 mm, 10 mm, and 20 mm between the top of the HFCCT experimental model and the bottom of the EPS. In each test, measurements of VEP on the top of the HFCCT experimental model and in other parts of the backfill soil, in addition to measuring the vertical displacement in the backfill soil, were taken after finishing the backfill process (see ).
Figure 23. Experimental test for using EPS geofoam in a combined horizontal and arch form as a method of VEP reduction on the HFCCT research experimental model.

Within the results of the experimental test results converted for the full-scale HFCCT research model, and present the relationships of the VEP and the percentage of reduction in the VEP on the HFCCT research model with the EPS thickness (EPS is in a combined horizontal and arch form) and the distance between the top of the HFCCT and the bottom of the EPS. For all the EPS thicknesses, as the thickness of the EPS increases, the vertical earth pressure on the HFCCT is reduced. The best result of vertical earth pressure reduction on the HFCCT was obtained when the EPS thickness is 3 m and the distance between the top of the HFCCT and the bottom of the EPS was 0.25 m, where the VEP on the HFCCT reduced from 716.6 kPa to 440.6 kPa (38.5% reduction in the VEP on the HFCCT) (see and ).
Figure 24. The relationship of the VEP on the HFCCT research model with the EPS thickness (in a combined horizontal and arch form) and the distance between the top of the HFCCT and the bottom of the EPS.

Figure 25. The relationship of the percentage of reduction in the VEP on the HFCCT research model with the EPS thickness (in a combined horizontal and arch form) and the distance between the top of the HFCCT and the bottom of the EPS.

The EPS geofoam inclusion in a combined horizontal and arch form leads the soil prism (interior prism) within the HFCCT structure width to settle more than the surrounding soil prisms (exterior prisms) due to deformation of the EPS (see ), the soil prism (interior prism) within the HFCCT structure width deform as a reverse arch-shape. Meanwhile, the reduced VEP on the HFCCT structure is equal to the shear force on the soil in the interior prism.
Figure 26. Measured vertical displacement of the backfill soil on the HFCCT research experimental model at a 90 mm backfill height in the experimental tests using EPS in a combined horizontal and arch form.

The results of the load reduction method using EPS geofoam in a combined horizontal and arch form indicate that the increase in distance between the EPS bottom and the top of the HFCCT does not enhance the soil arching effect but instead increases the VEP on the HFCCT. Therefore, the optimum distance can be considered the shortest distance between the EPS bottom and the top of the HFCCT.
The idea of using the EPS in a combined horizontal and arch form was to reduce more VEP on the HFCCT research experimental model by dissipating more VEP to the exterior soil prisms then to the side slopes of the valley through increasing the soil arching effect that forms in the backfill of the HFCCT. The results were acceptable but not good as expected.
5.4. Experimental tests for the HFCCT physical experimental model with load reduction method using EPS geofoam with the presence of geogrid on the top of the EPS in a horizontal form
18 tests were conducted using EPS geofoam with the presence of geogrid on the top of the EPS in a horizontal form on top of the HFCCT experimental model. In these tests, 6 EPS thicknesses of 10 mm, 20 mm, 30 mm, 40 mm, 50, and 60 mm were used with three distances of 5 mm, 10 mm, and 20 mm between the top of the HFCCT experimental model and the bottom of the EPS. In each test, measurements of VEP on the top of the HFCCT experimental model and in other parts of the backfill soil, in addition to measuring the vertical displacement in the backfill soil, were taken after finishing the backfill process (see ).
Figure 27. Experimental test for using EPS geofoam in a horizontal form with presence of geogrid above the EPS as a method of VEP reduction on the HFCCT research experimental model.

Within the results of the experimental test results converted for the full-scale HFCCT research model, and presents the relationships of the average VEP and the percentage of reduction in the VEP on the top of the HFCCT research model with the EPS thickness (EPS is in a horizontal form with the presence of geogrid on the top of the EPS) and the distance between the top of the HFCCT and the bottom of the EPS. For all the EPS thicknesses, as the thickness of the EPS increases, the vertical earth pressure on the HFCCT is reduced. The best result of vertical earth pressure reduction on the HFCCT was obtained when the EPS thickness is 3 m and the distance between the top of the HFCCT and the bottom of the EPS was 0.25 m, where the VEP on the HFCCT reduced from 716.6 kPa to 171.8 kPa (76.0% reduction in the VEP on the HFCCT) (see and ).
Figure 28. The relationship of the VEP on the HFCCT research model with the EPS thickness (in a horizontal form with presence of geogrid above the EPS) and the distance between the top of the HFCCT and the bottom of the EPS.

Figure 29. The relationship of the percentage of reduction in the VEP on the HFCCT research model with the EPS thickness (in a horizontal form with presence of geogrid above the EPS) and the distance between the top of the HFCCT and the bottom of the EPS.

The EPS geofoam inclusion in a horizontal form with the presence of geogrid on top of the EPS leads the soil prism (interior prism) within the HFCCT structure width to settle more than the surrounding soil prisms (exterior prisms) in a uniform and equal level due to deformation of the EPS and geogrid control of the EPS settlement and keep it in equal and uniform level (see ), the soil prism (interior prism) within the HFCCT structure width deform in equal and uniform level. Meanwhile, the reduced VEP on the HFCCT structure is equal to the magnitude of the shear force on the soil in the interior prism.
Figure 30. Measured vertical displacement of the backfill soil on the HFCCT research experimental model at a 90 mm backfill height in the experimental tests using EPS in a horizontal form with presence of geogrid above the EPS.

The results indicate that the increase in distance between the EPS bottom and the top of the HFCCT does not enhance the soil arching effect but instead increases the VEP on the HFCCT. Therefore, the optimum distance can be considered the shortest distance between the EPS bottom and the top of the HFCCT.
5.5. Experimental tests for the HFCCT physical experimental model with load reduction method using EPS geofoam with the presence of geogrid on the top of the EPS in a combined horizontal and arch form
18 tests were conducted using EPS geofoam with the presence of geogrid on the top of the EPS in a combined horizontal and arch form on top of the HFCCT experimental model. In these tests, 6 EPS thicknesses of 10 mm, 20 mm, 30 mm, 40 mm, 50, and 60 mm were used with three distances of 5 mm, 10 mm, and 20 mm between the top of the HFCCT experimental model and the bottom of the EPS. In each test, measurements of VEP on the top of the HFCCT experimental model and in other parts of the backfill soil, in addition to measuring the vertical displacement in the backfill soil, were taken after finishing the backfill process (see ).
Figure 31. Experimental test for using EPS geofoam in a combined horizontal and arch form with presence of geogrid above the EPS as a method of VEP reduction on the HFCCT research experimental model.

Within the results of the experimental test results converted for the full-scale HFCCT research model, and presents the relationships of the average VEP and the percentage of reduction in the VEP on the top of the HFCCT research model with the EPS thickness (EPS is in a combined horizontal and arch form with the presence of geogrid on the top of the EPS) and the distance between the top of the HFCCT and the bottom of the EPS. For all the EPS thicknesses, as the thickness of the EPS increases, the vertical earth pressure on the HFCCT is reduced. The best result of vertical earth pressure reduction on the HFCCT was obtained when the EPS thickness is 3 m and the distance between the top of the HFCCT and the bottom of the EPS was 0.25 m, where the VEP on the HFCCT reduced from 716.6 kPa to 245 kPa (65.8% reduction in the VEP on the HFCCT) (see and ).
Figure 32. The relationship of the VEP on the HFCCT research model with the EPS thickness (in a combined horizontal and arch form with presence of geogrid above the EPS) and the distance between the top of the HFCCT and the bottom of the EPS.

Figure 33. The relationship of the percentage of reduction in the VEP on the HFCCT research model with the EPS thickness (in a combined horizontal and arch form with presence of geogrid above the EPS) and the distance between the top of the HFCCT and the bottom of the EPS.

The EPS geofoam inclusion in a combined horizontal and arch form with the presence of geogrid on top of the EPS leads the soil prism (interior prism) within the HFCCT structure width to settle more than the surrounding soil prisms (exterior prisms) in a uniform and equal level due to deformation of the EPS and geogrid control of the EPS settlement and keep it in equal and uniform level (see ), the soil prism (interior prism) within the HFCCT structure width deform in equal and uniform level. Meanwhile, the reduced VEP on the HFCCT structure is equal to the magnitude of the shear force on the soil in the interior prism.
Figure 34. Measured vertical displacement of the backfill soil on the HFCCT research experimental model at a 90 mm backfill height in the experimental tests using EPS in a combined horizontal and arch form with presence of geogrid above the EPS.

The results of EPS geofoam inclusion in a combined horizontal and arch form with the presence of geogrid on top of the EPS as a load reduction method indicate that the optimum distance between the EPS bottom and the top of the HFCCT can be considered the shortest distance between the EPS bottom and the top of the HFCCT.
6. Conclusions
This study is to investigate and measure the coupled effect of using EPS and geogrid in two different formations on VEP reduction on HFCCTs due to soil arching and the relative vertical displacements of the HFCCT backfill soil prisms. Several influential factors, including the geogrid existence effect, the EPS formation, the EPS thickness, and the distance between the bottom of the EPS and the top of the HFCCT were studied. Therefore, the following conclusions can be drawn from this study:
The best result of EPS presence (without the presence of geogrid) on the top of an HFCCT is achieved using the EPS in a horizontal form as a method of VEP reduction on the HFCCT. This result is achieved by using 3.0 m EPS thickness and 0.25 m distance between the top of the HFCCT and the bottom of EPS, where the VEP on the HFCCT reduced from 716.6 kPa to 383.6 kPa (46.4% reduction in the VEP on the HFCCT).
The best result of EPS presence (with the presence of geogrid) on the top of an HFCCT is achieved using the EPS in a horizontal form as a method of VEP reduction on the HFCCT. This result is achieved by using 3.0 m EPS thickness and 0.25 m distance between the top of the HFCCT and the bottom of EPS, where the VEP on the HFCCT reduced from 716.6 kPa to 171.8 kPa (76.0% reduction in the VEP on the HFCCT).
Among the four scenarios of VEP reduction on the HFCCT used in this research, the best result was obtained using EPS in a horizontal form with the presence of geogrid above the EPS.
Disclosure statement
No potential conflict of interest was reported by the author(s).
Data availability statement
The manuscript has no associated data.
Additional information
Notes on contributors

Mohammad Hajiazizi
Mohammad Hajiazizi holds a Ph.D. in Geotechnical Engineering from the University of Shiraz, an M.Sc. in Geotechnical Engineering from the University of Tarbiat Modares, and a B.Sc. in Civil Engineering from the University of Tabriz, Iran. As an Associate Professor in the Civil Engineering Department at the Faculty of Engineering, Razi University, Iran. His research interests span a wide spectrum within Geotechnical Engineering, including Numerical Modeling, Computer Codes for Geotechnics, Machine Learning in Geotechnics, Physical Modeling in Geotechnics, Laboratory Tests in Static and Cyclic States, and Slope Stability Analysis in Rock and Soil Mechanics. Dr. Hajiazizi has authored over 70 papers published in esteemed journals in Geotechnical engineering, including ASCE, Elsevier, Springer, and Taylor, among others. Additionally, he has actively participated in more than 30 national and international congresses, showcasing his commitment to advancing the field through scholarly discourse and collaboration.
References
- Abdollahi, M., Tafreshi, S. N. M., & Leshchinsky, B. (2019). Experimental-numerical assessment of geogrid-EPS systems for protecting buried utilities. Geosynthetics International, 26(4), 333–353. https://doi.org/10.1680/jgein.19.00013
- Abdullah, C. H., & Edil, T. B. (2007). Behaviour of geogrid-reinforced load transfer platforms for embankment on rammed aggregate piers. Geosynthetics International, 14(3), 141–153. https://doi.org/10.1680/gein.2007.14.3.141
- Abu-Farsakh, M. Y., Chen, Q., & Yoon, S. (2008). Use of Reinforced Soil Foundation (RSF) to Support Shallow Foundation.
- Adams, M. T., & Collin, J. G. (1997). Large model spread footing load tests on geosynthetic. Journal of Geotechnical and Geoenvironmental Engineering, 123(1), 66–72. https://doi.org/10.1061/(ASCE)1090-0241(1997)123:1(66)
- Al-Barqawi, M., Aqel, R., Wayne, M., Titi, H., & Elhajjar, R. (2021). Polymer geogrids: A review of material, design and structure relationships. Materials, 14(16), 4745. https://doi.org/10.3390/ma14164745
- Alotaibi, E., Omar, M., Shanableh, A., Zeiada, W., Fattah, M. Y., Tahmaz, A., & Arab, M. G. (2021). Geogrid bridging over existing shallow flexible PVC buried pipe – Experimental study. Tunnelling and Underground Space Technology, 113(March), 103945. https://doi.org/10.1016/j.tust.2021.103945
- Arkawazi, S. A. F., & Hajiazizi, M. (2022). Using expanded polystyrene geofoam and tire-derived aggregate in different forms to reduce vertical earth pressure on high-filled cut-and-cover tunnels. Cogent Engineering, 9(1), 0–32. https://doi.org/10.1080/23311916.2022.2138100
- Arkawazi, S. A. F., & Hajiazizi, M. (2023). Coupled effect of tire-derived aggregate and geogrid on lateral earth pressure on high-filled cut-and-cover tunnels. 1, 73–87. https://doi.org/10.22059/IJMGE.2022.346697.594987
- Bathurst, R. J., Zarnani, S., & Gaskin, A. (2007). Shaking table testing of geofoam seismic buffers. Soil Dynamics and Earthquake Engineering, 27(4), 324–332. https://doi.org/10.1016/j.soildyn.2006.08.003
- Bazzazian Bonab, S., Lajevardi, S. H., Saba, H. R., & Mirhosseini, S. M. (2021). The novel usage of EPS Geofoam as column material: A laboratory study. International Journal of Geosynthetics and Ground Engineering, 7(1), 1–14. https://doi.org/10.1007/s40891-020-00252-9
- Beju, Y. Z., & Mandal, J. N. (2017a). Combined use of jute geotextile-EPS geofoam to protect flexible buried pipes: experimental and numerical studies. International Journal of Geosynthetics and Ground Engineering, 3(4), 1–20. https://doi.org/10.1007/s40891-017-0107-5.
- Beju, Y. Z., & Mandal, J. N. (2017b). Expanded Polystyrene (EPS) Geofoam: Preliminary characteristic evaluation. Procedia Engineering, 189(May), 239–246. https://doi.org/10.1016/j.proeng.2017.05.038
- Brown, S. F., Kwan, J., & Thom, N. H. (2007). Identifying the key parameters that influence geogrid reinforcement of railway ballast. Geotextiles and Geomembranes, 25(6)’, 326–335. https://doi.org/10.1016/j.geotexmem.2007.06.003
- Chun, B. S., Lim, H.-S., Sagong, M., & Kim, K. (2004). Development of a hyperbolic constitutive model for expanded polystyrene (EPS) geofoam under triaxial compression tests. Geotextiles and Geomembranes, 22(4), 223–237. https://doi.org/10.1016/j.geotexmem.2004.03.005
- Dong, Y., Han, J., & Bai, X. (2010). Numerical analysis of tensile behavior of geogrids with rectangular and triangular apertures. Geotextiles and Geomembranes, 29(2), 83–91. https://doi.org/10.1016/j.geotexmem.2010.10.007
- Dugkov, M. (1998). Materials Research on EPS20 and EPS15 Under Representative Conditions in Pavement Structures. Geotextiles and Geomembranes, 15(1997), 147–181.
- Dugkov, M., & Scarpas, A. (1998). Three-Dimensional Finite Element Analysis of Flexible Pavements with an (Open Joint in the) EPS Sub-Base. Geotextiles and Geomembranes, 15(1997), 29–38.
- Fakhraldin, M. K. (2012 Measurement of tensile properties of geogrids [Paper presentation]. Second International Conference on Geotechnique, Construction Materials and Environmen.
- Farnsworth, C. B., Bartlett, S. F., Negussey, D., & Stuedlein, A. W. (2008). Rapid construction and settlement behavior of embankment systems on soft foundation soils. Journal of Geotechnical and Geoenvironmental Engineering, 134(3), 289–301. https://doi.org/10.1061/_ASCE_1090-0241_2008_134:3_289
- Fattah, M. Y., Zbar, B. S., & Al-Kalali, H. H. M. (2023). Experimental investigation of the performance of buried flexible pipe in reinforced sand. Slovak Journal of Civil Engineering, 31(2), 48–60. https://doi.org/10.2478/sjce-2023-0012
- Gade, V. K., & Dasaka, S. M. (2022). Short- and long-term behavior of EPS geofoam in reduction of lateral earth pressure on rigid retaining wall subjected to surcharge loading. Geotextiles and Geomembranes, 50(5), 868–880. https://doi.org/10.1016/j.geotexmem.2022.05.002
- Gao, H., Chen, G., & Wang, Z. (2011). The mechanical behaviors of the embankment filled with EPS composite soil. Advanced Materials Research, 368-373, 2813–2818. https://doi.org/10.4028/www.scientific.net/AMR.368-373.2813
- Gao, H., Liu, J., & Liu, H. (2011). Geotechnical properties of EPS composite soil. International Journal of Geotechnical Engineering, 5(1), 69–77. https://doi.org/10.3328/IJGE.2011.05.01.69-77
- Gu, A. Q., Guo, T. T., & Wang, X. P. (2005). Experimental study on reducing load measurement using EPS of culvert under high-stacked soil. Chinese Journal of Geotechnical Engineering, 27(5), 2005.
- Han, J., & Akins, K. (2002). Use of geogrid-reinforced and pile-supported earth structures. Deep Foundations, 0, 668–679. https://doi.org/10.1061/40601(256)48
- Han, J., & Leshchinsky, D. (2010). Analysis of back-to-back mechanically stabilized earth walls. Geotextiles and Geomembranes, 28(3), 262–267. https://doi.org/10.1016/j.geotexmem.2009.09.012
- Hassan, D., Saidani, M., & Shibani, A. (2021). Behaviour of a Foam Mixture as a Lightweight Construction Material. International Journal of Geosynthetics and Ground Engineering, 7(3), 1–9. https://doi.org/10.1007/s40891-021-00296-5
- Hatami, K., & Witthoeft, A. F. (2008). A numerical study on the use of geofoam to increase the external stability of reinforced soil walls. Geosynthetics International, 15(6), 452–470. https://doi.org/10.1680/gein.2008.15.6.452
- Hazarika, H. (2006). Stress – strain modeling of EPS geofoam for large-strain applications. Geotextiles and Geomembranes, 24(2), 79–90. https://doi.org/10.1016/j.geotexmem.2005.11.003
- Helstrom, C. L., Humphrey, D. N., & Hayden, S. A. (2006 Geogrid Reinforced Pavement Structure in a Cold Region [Paper presentation]. Cold Regions Engineering, (401), pp. 1–12. https://doi.org/10.1061/40836(210)57
- Horvath, J. S. (1998). The Compressible Inclusion Function of EPS Geofoam. Geotextiles and Geomembranes, 15(1-3), 77–120. https://doi.org/10.1016/S0266-1144(97)00008-3
- Horvath, J. S. (2004 Geofoam Compressible Inclusions: The New Frontier In Earth Retaining Structures [Paper presentation].Asce,. https://doi.org/10.1061/40744(154)187
- Huang, J., & Han, J. (2009). 3D coupled mechanical and hydraulic modeling of a geosynthetic-reinforced deep mixed column-supported embankment. Geotextiles and Geomembranes, 27(4), 272–280. https://doi.org/10.1016/j.geotexmem.2009.01.001
- Huang, X., & Negussey, D. (2011). EPS geofoam design parameters for pavement structures.
- Jili, Q., Huan, T., Weiqing, Q., Guoqi, H., Hongmei, L., Abulimiti, P., Maimaitiyusupu, S., & Batugin, A. (2022). Modification of mechanical properties of Shanghai clayey soil with expanded polystyrene. Science and Engineering of Composite Materials, 29(1), 37–49. https://doi.org/10.1515/secm-2022-0004
- Jutkofsky, W. S., Sung, J. T., & Negussey, D. (2000). Stabilization of Embankment Slope with Geofoam. Transportation Research Record: Journal of the Transportation Research Board, 1736(1), 94–102. https://doi.org/10.3141/1736-12
- Kang, J., Parker, F., & Yoo, C. H. (2007). Soil-Structure Interaction and Imperfect Trench Installations for Deeply Buried Concrete Pipes. Journal of Geotechnical and Geoenvironmental Engineering, 133(3), 277–285. https://doi.org/10.1061/(asce)1090-0241(2007)133:3(277)
- Khan, M. I., & Meguid, M. A. (2018). Experimental investigation of the shear behavior of EPS geofoam. International Journal of Geosynthetics and Ground Engineering, 4(2), 0. https://doi.org/10.1007/s40891-018-0129-7
- Khan, M. I., & Meguid, M. A. (2021). A Numerical study on the role of EPS geofoam in reducing earth pressure on retaining structures under dynamic loading. International Journal of Geosynthetics and Ground Engineering, 7(3) https://doi.org/10.1007/s40891-021-00304-8
- Khan, M. R., & Dasaka, S. M. (2020a). Amplification of vibrations in high-speed railway embankments by passive ground vibration barriers. International Journal of Geosynthetics and Ground Engineering, 6(3), 1–15. https://doi.org/10.1007/s40891-020-00217-y
- Khan, M. R., & Dasaka, S. M. (2020b). EPS geofoam as a wave barrier for attenuating high-speed train-induced ground vibrations: A single-wheel analysis. International Journal of Geosynthetics and Ground Engineering, 6(4) https://doi.org/10.1007/s40891-020-00230-1
- Khodayari, A. H., & Dabiri, R. (2018). Effects of geogrid layers on improving bearing capacity of vibrating machines foundation. Journal of New Approaches in Civil Engineering, 2(2), 11–26.
- Kinney, T. C., Danielle, K. S., & Schuler, J. (1998). Using geogrids for base reinforcement as measured by falling weight deflectometer in full-scale laboratory study. Transportation Research Record: Journal of the Transportation Research Board, 1611(1), 70–77. https://doi.org/10.3141/1611-09
- Koerner, R. (2005). Designing with Geosynthetics (5th edn.). Pearson Prentice Hall.
- Leo, C. J., Kumruzzaman, M., Wong, H., & Yin, J. H. (2008). Behavior of EPS geofoam in true triaxial compression tests. Geotextiles and Geomembranes, 26(2), 175–180. https://doi.org/10.1016/j.geotexmem.2007.10.005
- Li, S., Ho, I.-H., Ma, L., Yao, Y., & Wang, C. (2019). Load reduction on high-filled cut-and-cover tunnel using discrete element method. Computers and Geotechnics, 114(March), 103149. https://doi.org/10.1016/j.compgeo.2019.103149
- Li, S., Han, G., Ho, I.-H., Ma, L., Wang, Q., & Yu, B. (2020). Coupled effect of cross-sectional shape and load reduction on high-filled cut-and-cover tunnels considering soil–structure interaction. International Journal of Geomechanics, 20(7), 04020082. https://doi.org/10.1061/(asce)gm.1943-5622.0001696
- Li, S., Yao, Y., Ho, I.-H., Ma, L., Wang, Q., & Wang, C. (2020). Coupled effect of expanded polystyrene and geogrid on load reduction for high-filled cut-and-cover tunnels using the discrete-element method. International Journal of Geomechanics, 20(6), 04020052. https://doi.org/10.1061/(asce)gm.1943-5622.0001683
- Li, S., Jianie, Y., Ho, I.-H., Ma, L., Wang, Q., & Yu, B. (2020). Experimental and numerical analyses for earth pressure distribution on high-filled cut-and-cover tunnels. KSCE Journal of Civil Engineering, 24(6), 1903–1913. https://doi.org/10.1007/s12205-020-1693-7
- Marston, A., & Anderson, A. O. (1913). The theory of loads on pipes in ditches and tests of cement and clay drain tile and sewer pipe. Iowa Engineering Experiment Station, Iowa State College. https://doi.org/10.1785/gssrl.8.3.1a
- Marston, M. G. (1930). The theory of external loads on closed conduits in the light of the latest experiments. Highway Research Board Proceeding, 9, 138–170. https://trid.trb.org/view/103945
- McAffee, R. P., & Valsangkar, A. J. (2008). Field performance, centrifuge testing, and numerical modelling of an induced trench installation. Canadian Geotechnical Journal, 45(1), 85–101. https://doi.org/10.1139/T07-086
- McGuigan, B. L., & Valsangkar, A. J. (2010). Centrifuge testing and numerical analysis of box culverts installed in induced trenches. Canadian Geotechnical Journal, 47(2), 147–163. https://doi.org/10.1139/T09-085
- Meguid, M. A., Ahmed, M. R., Hussein, M. G., & Omeman, Z. (2017). Earth Pressure Distribution on a Rigid Box Covered with U-Shaped Geofoam Wrap. International Journal of Geosynthetics and Ground Engineering, 3(2), 0. https://doi.org/10.1007/s40891-017-0088-4
- Meguid, M. A., & Ahmed, M. R. (2020). Earth pressure distribution on buried pipes installed with geofoam inclusion and subjected to cyclic loading. International Journal of Geosynthetics and Ground Engineering, 6(1), 1–8. https://doi.org/10.1007/s40891-020-0187-5
- Meguid, M. A., & Hussein, M. G. (2017). A numerical procedure for the assessment of contact pressures on buried structures overlain by EPS geofoam inclusion. International Journal of Geosynthetics and Ground Engineering, 3(1), 1–14. https://doi.org/10.1007/s40891-016-0078-y
- Murashev, A., Easton, M., & Kathirgamanathan, P. (2013). Advanced Numerical Modelling of Geogrid-reinfroced Rockfall Protection Embankments [Paper presentation]. Proceedings 19th NZGS Geotechnical Symposium.
- Nawghare, S. M., & Mandal, J. N. (2020). Effectiveness of Expanded Polystyrene (EPS) beads size on fly ash properties. International Journal of Geosynthetics and Ground Engineering, 6(1), 1–11. https://doi.org/10.1007/s40891-020-0189-3
- Ossa, A., & Romo, M. P. (2009). Micro- and macro-mechanical study of compressive behavior of expanded polystyrene geofoam. Geosynthetics International, 16(5), 327–338. https://doi.org/10.1680/gein.2009.16.5.327
- Ossa, A., & Romo, M. P. (2011). Dynamic characterization of EPS geofoam. Geotextiles and Geomembranes, 29(1), 40–50. https://doi.org/10.1016/j.geotexmem.2010.06.007
- Padade, A. H., & Mandal, J. N. (2012). Behavior of expanded polystyrene (EPS) geofoam under triaxial loading conditions. Electronic Journal of Geotechnical Engineering, 17 S, 2542–2553.
- Qingbiao, W., Cong, Z., Tiantian, W., Yun, B., Rongshan, L. Ü., Lei, X., Junxian, Z., Zhongjing, H., Fei, X., Tao, Z., Zhen, C., Lingyu, T., & Hui, W. (2015). The mechanical property of bidirectional geogrid and its application research in retaining wall design. The Open Construction and Building Technology Journal, 9(1), 214–222. https://doi.org/10.2174/1874836801509010214
- Ram Rathan Lal, B., & Nawkhare, S. S. (2016). Experimental study on plastic strips and EPS beads reinforced bottom ash based material. International Journal of Geosynthetics and Ground Engineering, 2(3), 1–12. https://doi.org/10.1007/s40891-016-0066-2
- Rodríguez, L. M., Arroyo, M., & Cano, M. M. (2018). Use of tire derived aggregate in tunnel cut-and-cover. Canadian Geotechnical Journal, 55(7), 968–978. https://doi.org/10.1139/cgj-2017-0446
- Rousé, P. C. (2018). Numerical modeling and analysis of pullout tests of sheet and geogrid inclusions in sand. The university of British Columbia.
- Shafikhani, A., Bheemasetti, T. V., & Puppala, A. J. (2017). Effect of seasonal changes on a hybrid soil–geofoam embankment system. International Journal of Geosynthetics and Ground Engineering, 3(4),. https://doi.org/10.1007/s40891-017-0116-4
- Sinmez, B. (2019). Characterization of Geogrid Reinforced Ballast Behavior Through Finite Element Modeling, Scholar Commons. University of South Florida.
- Sladen, J. A., & Oswell, J. M. (1988). The induced trench method - a critical review and case history. Canadian Geotechnical Journal, 25(3), 541–549. https://doi.org/10.1139/t88-059
- Spangler, M. G. (1958 A practical application of the imperfect ditch method of construction [Paper presentation]. 37th Annual Meeting of the Highway Research Board), Highway Research Board (pp. 271–277).
- Sravanam, P. P., & Mouli, S. (2022). Optimum Orientation of Geofoam Inclusion in the Retaining Wall Optimum Orientation of Geofoam Inclusion in the Retaining. Advancements in Sustainable Materials and Infrastructure, 1–9. https://doi.org/10.1088/1755-1315/1086/1/012016
- Stark, T. D., Arellano, D., & Horvath, J. S. (2004). Geofoam Applications in the Design and Construction of Highway Embankments, Prepared for : Submitted by : Urbana, Illinois. Transportation Research Board of The National Academies.
- Tang, X., Chehab, G. R., & Palomino, A. (2008). Evaluation of geogrids for stabilising weak pavement subgrade. International Journal of Pavement Engineering, 9(6), 413–429. https://doi.org/10.1080/10298430802279827
- Taylor, R. K. (1973). Induced-trench method of culvert installation. Highw Research Record, 443, 15–31.
- Tharani, K., Mahendran, N., & Vijay, T. J. (2019). Experimental investigation of geogrid reinforced concrete slab. International Journal of Engineering and Advanced Technology, 8(3S), 158–163.
- Unipod. (2021). Unibloc GeoFoam by Unipod. Truganina, Vic 3029 Australia: Unipod. https://www.unipod.com.au/.
- Vaslestad, J., Johansen, T. H., & Holm, W. (1993). Load reduction on rigid culverts beneath high fills: long-term behavior. Transportation Research Record, 1415, 58–68.
- Vorobjovas, V., & Vaitkus, A. (2017). 3D-FEM analysis on geogrid reinforced flexible pavement roads 3D-FEM analysis on geogrid reinforced flexible pavement roads. Earth and Environmental Science, 95, 022024. https://doi.org/10.1088/1755-1315/95/2/022024
- Wong, H., & Leo, C. J. (2006). A simple elastoplastic hardening constitutive model for EPS geofoam. Geotextiles and Geomembranes, 24(5), 299–310. https://doi.org/10.1016/j.geotexmem.2006.03.007
- Wood, D. M. (2017). Geotechnical modelling, Geotechnical Modelling. https://doi.org/10.1201/9781315273556
- Xian Yima Optoelec Limited Company. (2022). DM-YB1808/20/40/60/80 Dynamic and Static Strain Test System (pp. 1–7). Xian Yima Optoelec Co., Ltd.
- Xiaoming, Y. (2012). An assessment of the geometry effect of geosynthetics for base course reinforcements. International Journal of Transportation Science and Technology, 1(3), 247–257. https://doi.org/10.1260/2046-0430.1.3.247
- Xie, M., Zheng, J., Shao, A., Miao, C., & Zhang, J. (2020). Study of lateral earth pressures on nonyielding retaining walls with deformable geofoam inclusions. Geotextiles and Geomembranes, 48(5), 684–690. https://doi.org/10.1016/j.geotexmem.2020.05.003
- Zarnani, S., & Bathurst, R. J. (2007). Experimental investigation of EPS geofoam seismic buffers using shaking table tests. Geosynthetics International, 14(3), 165–177. https://doi.org/10.1680/gein.2007.14.3.165
- Zarnani, S., & Bathurst, R. J. (2008). Numerical modeling of EPS seismic buffer shaking table tests. Geotextiles and Geomembranes, 26(5), 371–383. https://doi.org/10.1016/j.geotexmem.2008.02.004
- Zhou, W-h., Lao, J-y., Huang, Y., & Chen, R. (2016). Three-dimensional finite element modelling of soil arching in pile-supported geogrid-reinforced embankments. Procedia Engineering, 143(Ictg), 607–614. https://doi.org/10.1016/j.proeng.2016.06.081
- Zhuo, B., Wang, F., Fang, Y., Chen, Y., & Ning, G. (2020). Analysis of cracking development and mechanical characteristics of high-filled cut-and-cover tunnel. KSCE Journal of Civil Engineering, 24(8), 2519–2532. https://doi.org/10.1007/s12205-020-0247-3