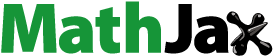
Abstract
This paper presents an experimental and numerical investigation of RCC (Reinforced Concrete) piles with helical grooves under axial and lateral loads in cohesionless soil. A set of experiments is carried out to assess the performance of RCC piles with helical grooves. To replicate the experimental findings, the Finite Element Method (FEM) is employed using Abaqus software. Three-dimensional (3D) models are created to represent both the pile and the soil using Abaqus. The paper compares settlement characteristics and lateral displacement of plain RCC piles and RCC piles with helical grooves. This study also evaluates the impact of varying the pitch of helical grooves on enhancing pile performance. The results indicate that RCC piles with helical grooves outperform plain RCC piles in terms of both axial and lateral load-carrying capacity, inferred from both experimental and analytical techniques.
REVIEWING EDITOR:
1. Introduction
Pile foundations are a very popular foundation system used to transfer loads from the superstructure of a building or structure to the underlying soil. Piles are commonly used in deep foundation systems where the soil is too weak to support the load of the structure on its own. In the design of pile foundations, it is crucial to consider their response to both vertical and lateral loads. Understanding the interaction between the pile and the surrounding soil is also essential for effective pile design.
There are several types of piles normally used for various infrastructure projects, viz. driven piles, drilled piles, and helical piles. The effectiveness of RCC piles in different types of soil for resisting axial and lateral loading has been previously studied by many researchers (Lu et al., Citation2023; Ooi et al., Citation2004; Saha et al., Citation2020; Zheng et al., Citation2023). RCC piles are used widely, because of ease in manufacturing and have proven to be economical compared to the steel helical pile. However, RCC piles typically require more time for installation compared to steel helical piles and during the installation of RCC piles, the soil around the pile is disturbed due to excavation and pouring of concrete. This disturbance can affect the surrounding soil properties and potentially may lead to settlement issues. Steel helical pile is another type of commonly used pile, wherever the foundation encounters a weak and requires higher load-carrying capacity. The steel helical piles possess higher load-carrying capacity as compared to RCC piles due to the presence of helices over the surface of the pile. Each of these helices provides bearing support wherever they are located along with anchoring action when the pile is driven (Bao et al., Citation2022; Chen et al., Citation2020; Choo, Citation2018; Dehestani & Mousavi, Citation2015; IS:2911 (Part 1/Section 2), Citation2010; Poulos, Citation2018; Rao & Prasad, Citation1993; Soltani-Jigheh & Zahedi, Citation2020; Srijaroen et al., Citation2021; Taborda et al., Citation2022; Venkatesan & Mayakrishnan, Citation2022; Vignesh & Muthukumar, Citation2023). The installation of steel helical piles is easier and produces lower vibrations and noise (Choi et al., Citation2013; Da Silva et al., Citation2023; Zarrabi & Eslami, Citation2016; Zhou et al., Citation2023).
The helical piles are generally made of steel, which is more economical than RCC piles for similar load conditions. Moreover, steel helical piles also encounter a problem with corrosion in harsh environments. The corrosion of steel helical piles degrades the strength of the pile resulting in lower load-carrying capacity (Liu et al., Citation2022; Shen et al., Citation2023; Yang et al., Citation2022). To minimize the drawbacks of steel helical piles and RCC piles, a novel pile design is used in the present study with the inclusion of helical grooves. Introducing helical grooves to the RCC piles will bring together the beneficial effects of helical grooves and RCC as core material. The RCC pile with helical grooves can be driven by applying torque at the top of the pile, in contrast to the plain RCC pile. This torque application method is typically used to install helical piles, which can be twisted or ‘screwed’ into the ground, making them an efficient and practical choice for certain applications. In contrast, plain RCC piles are usually driven into the ground using methods like impact driving or vibratory techniques (Lu et al., Citation2023). Incorporating helical grooves into a circular RCC pile enhances its surface area for a similar driving length enabling the helical grooves to interlock with the soil at various pitch intervals along with the anchoring effect (Mittal et al., Citation2010). Each helical groove offers end bearing effect at various pitch intervals, impacting a greater volume of cohesionless soil upon loading, resulting in an increase in the axial and lateral load-carrying capacity.
Despite the potential benefits offered by RCC and steel helical piles, there has been limited research conducted on RCC piles with helical grooves. The objective of this study is to address this gap by examining the performance of RCC piles with helical grooves using a combination of experimental and numerical approaches. Numerical analysis is carried out with the FE software Abaqus, which allows the modelling and simulation of both the pile and the surrounding soil under different loading scenarios. The material properties, boundary conditions, and applied loads, along with the interaction between the pile and the soil can be accurately represented by incorporating interface elements (Abbasa et al., Citation2015; Baqi et al., Citation2022; Chen et al., Citation2022; Jawad & Han, Citation2021; Khodair & Abdel-Mohti, Citation2014; Li et al., Citation2022; Nowkandeh & Choobbasti, Citation2021; Zhang & Zhuo, Citation2014).
2. Materials and methods
2.1. Test specimen
For the present study, concrete with a slump of 250 mm was used to cast the specimens. Concrete with high fluidity was required since the concrete must pass through the complex geometry of the mould with helices. Galvanized iron moulds are used to cast the test specimens. According to IS 2911: Part 1: Sec 2 and Sec 3: 2010, the minimum grade of concrete for precast piles is M25, hence in the present study M30 grade of concrete is used. The minimum area of steel recommended as per the same code is 0.4% of the cross-sectional area of the pile, hence in the current study, are of steel used was 1% of the cross-sectional area of the pile. The target strength of the concrete mix was 38.25 N/mm2 at 28 days, while the actual ultimate compressive strength achieved was 38 N/mm2. illustrates the mix proportions used in the study.
Table 1. Concrete mix highlights.
The process of pile casting is shown in . In the first stage, the mould is prepared for concrete, and in the second stage the prepared concrete is poured into the mould and after 24 h the specimens are de-moulded and kept for curing. However, the detailed explanation of the process of pile casting is explained in the subsequent paragraphs.
The rubber tube of a square cross-section of size 10 × 10 mm with a hardness of 69 shores is adhered to an inner surface of the mould to represent the helices of the pile as shown in .
The inner surface of the mould is lubricated and the steel rebar of 8 mm diameter and grade Fe500 is placed centrally in the mould as shown in for plain piles, similarly, for piles with helical groves, the steel rebar is placed centrally.
The concrete is then poured inside the mould and left for setting. After 24 h of casting the demoulding of the test specimens is carried out, shows the demoulding of the specimen and shows the final test specimen.
Figure 4. (a) Demoulding of the pile with helical groove specimen. (b) Pile with the helical groove test specimen.

For the axial and lateral loading performance of the pile, four piles of different pitch lengths are cast, as shown in . These are as follows (a) is a control pile without a helical groove denoted as (P0), (b) is an RCC pile with a helical groove of pitch 50 mm (P50), (c) is an RCC pile with helical groove of pitch 75 mm (P75) and (d) is RCC pile with helical groove of pitch 100 mm (P100).
Figure 5. (a) Control pile P0, (b) RCC pile with helical grooves P50, (c) RCC pile with helical grooves P75, and (d) RCC pile with helical grooves P100.

All piles are identical in length and diameter, the pile length is 800 mm (L) and diameter 80 mm (D), hence the L/D ratio of the pile is 10 and the helical groove size is 10 × 10 mm, the groove depth to diameter ratio is considered as 0.125 (Chen et al., Citation2020). Hence the full-scale piles are replicated in the ratio of L/D and groove depth to diameter of the pile to provide a similar effect as laboratory scale. For each of the piles tested for axial and lateral load, the load vs. settlement characteristics and lateral displacements are recorded.
2.2. Axial and lateral loading test setup
Axial load testing is a common method used to evaluate the performance of piles under compressive loads. This test involves applying a vertical load to the pile while monitoring its deformation and the resistance it offers to the load.
In the present study, the lever arm concept is employed for the application of axial loading (Hassan et al., Citation2019), which eliminates the higher dead weight requirement for the reaction. The test setup consists of a brick masonry tank of 1250 (L) × 1250 (W) × 1500 (D) mm inner dimensions. The loading frame is made up of a steel C section of size 100 × 50 × 5 mm as shown in . shows the loading setup with the location of dial gauges to measure the settlement of the pile.
Figure 6. The axial load test setup (a) line diagram of the testing tank and (b) dial gauges, sand, and pile.

In the present study, cohesionless soil was used in the tank for testing the axial and lateral load performance of the RCC pile with helical grooves. Sand of fixed volume of 1.9 m³ is filled into the tank. This cohesionless soil is added in three layers, with each layer being 0.4 m thick and a volume of 0.625 m3. Each layer is compacted to achieve this fixed volume. For this, 1000 kg of sand is compacted using an electric needle vibrator until the sand level reaches a height of 0.4 m from the bottom of the tank. This compaction process results in the sand achieving a relative density of 85%. Chen et al. (Citation2020) also adopted a similar procedure to compact the sand in the testing tank. All the physical properties of cohesionless soil are obtained by experimental methods as per standards (IS 2386-Part III, Citation1963; 2720: Part 13, Citation1986; 2720: Part 14, Citation1983; 2720: Part 4, Citation1985) and are presented in .
Table 2. Physical properties of cohesionless soil.
Two dial gauges with the least count of 0.01 mm are used to measure the settlement in axial loading and for lateral loading, one dial gauge is used to record the displacement of the pile for each load increment. In pile testing, load increment measurement is a common technique used to evaluate the pile’s load-carrying capacity and deformation behaviour under increasing loads. The load increment process involves applying a series of load increments on the pile and measuring the corresponding pile response at each load increment. Each load increment was held constant for 2 h to allow the pile response to stabilize to a limit of dial gauge reading, not more than 0.2 mm. The magnitude of the load increment can vary depending on the specific testing protocol. However, in this study, 20% of the safe load is considered for testing a pile.
A lateral load test of a pile is conducted to determine the pile’s capacity to resist lateral loads, that act perpendicular to the pile’s axis. This type of test is often performed on foundation piles that support structures, such as bridges, buildings, and transmission towers. During the lateral load test, a hydraulic jack or any other load cell is used to apply a horizontal load to the pile at a predetermined level above the ground. The load is gradually increased until the pile reaches its ultimate lateral capacity or until an allowable deflection limit is reached (IS 2911 (Part 4), Citation2013). Measurements of the pile’s lateral deflection and the applied load are recorded during the test, and this data is used to construct the load-deflection curve. The load-deflection curve can be used to determine the pile’s lateral stiffness and ultimate capacity.
In this study lateral loading is applied with the help of a steel rope wrapped around the circumference of the pile. The rope is made to pass over a pulley, installed on one of the side walls of the tank. The other end of the rope is left suspended, and the load is applied at that suspended end. The lateral load setup is shown in . shows the steel rope with the location of the dial gauge and pile.
Figure 7. The lateral load test setup (a) line diagram of the setup and (b) steel rope, dial gauge, sand, and pile.

The load increment used in both axial and lateral load applications is 20% of the safe axial and lateral load (IS 2911 (Part 4), Citation2013). In practice, the piles are driven into the soil media, however, in the present experimental setup, the pile is installed first in position as suggested by (Chen et al., Citation2018, Citation2020; Soltani-Jigheh & Zahedi, Citation2020), since the actual driving of the pile in laboratory setup was not practicable. The cohesionless soil is later poured into the tank, layer by layer, and compacted to a desired relative density of 85%.
2.3. Numerical simulation
An Abaqus tool is used to simulate the behaviour of axial and lateral loading of the pile with the surrounding soil.
As per , the pile is modelled as a deformable body with linear elastic properties using C3D10M: A 10-noded modified quadratic tetrahedron element. The element’s shape and connectivity allow for efficient modelling of complex geometries, it can capture both linear and nonlinear deformations, and the cohesionless soil is modelled as an elastoplastic Mohr-Coulomb continuum model using C3D8R: A flexible 8-node linear brick element incorporating hourglass control and reduced integration is used. Unlike an element employing full integration, this element retains its flexibility during bending. The integration point, positioned at the element’s centre, ensures the most precise stress and strain measurements at the integration points. By implementing hourglass control, zero energy modes are eliminated effectively, preventing significant displacements from interfering with the accurate solution (George et al., Citation2020; Khodair & Abdel-Mohti, Citation2014; Mardfekri et al., Citation2013). The mechanical properties of the cohesionless soil and pile are shown in .
Table 3. Mechanical properties of cohesionless soil and pile.
The cohesionless soil is modelled as a 3D elastoplastic Mohr-Coulomb model of size 1250 (L) × 1250 (W) × 1200 (D) mm to simulate the experimental setup as shown in . Generally, sand is treated as a cohesionless material, where c = 0. In the classical Mohr–Coulomb model presented, the friction angle and dilatancy angle are considered constant material parameters. However, it’s important to note that these parameters can vary based on the initial relative density and stress level of the sand being modelled. The specific values of these angles are influenced by the conditions of the sand material, with variations in density and stress level leading to adjustments in the friction and dilatancy angles in the model (Abusharar et al., Citation2009; Choo, Citation2018; Geotechnica & Do, Citation2016; Taborda et al., Citation2022).
The interaction between soil and pile is modelled as friction contact by using the penalty method, by defining the tangential and normal contact behaviour in the finite element model (Khodair & Abdel-Mohti, Citation2014). The master surface is defined as the pile’s external surface and the slave surface is the soil’s internal surface, which was extruded to match the pile dimensions for the contact as shown in (George et al., Citation2020; Khodair & Abdel-Mohti, Citation2014), the penalty method permits relative motion of the surfaces as elastic slip (Ali et al., Citation2016). For the interaction between soil and pile, the tangential friction coefficient is considered as 0.46 which is (2/3 of ɸ) for the finite element model (Chiou & Wei, Citation2021).
2.3.1. Mesh dependency test
Mesh dependency in finite element software pertains to how sensitive simulation results are to the mesh’s size and quality. The mesh size significantly influences simulation outcomes. A coarser mesh yields faster computation but less accurate results, whereas a finer mesh leads to more accurate results but requires a longer computational time. In this study, various mesh sizes were examined to determine their impact on the test results. It was observed that at mesh sizes of 50 mm number of elements is 29,104, and at 25 mm number of elements is 186,028, the recorded Von Mises stress in the soil just below the pile end, values did not exhibit significant variation, as indicated in . Given the available computational resources, a 50 mm mesh size was chosen for this study due to its balance between accuracy and computational efficiency.
The soil side faces are restrained in horizontal directions by using displacement control, and the bottom face of the soil is fully restrained in all directions as shown in (Abusharar et al., Citation2009; Khodair & Abdel-Mohti, Citation2014).
The geostatic procedure is typically the initial phase of a geotechnical analysis. During this step, gravity loads are applied as shown in . Ideally, in this phase, the applied loads and initial stresses should be carefully balanced to achieve an equilibrium, resulting in zero deformations. This approach ensures that the system is initially stable and ready for further analysis and evaluation. All the elements of the pile and degrees of freedom are restrained to a reference point to make it behave like a rigid body when it is loaded as a guided directional movement (Khodair & Abdel-Mohti, Citation2014). The load is applied as a concentrated load at the reference point, acting down words for axial load and horizontal for lateral load simulation, with each increment as 20% of the safe load.
3. Results and discussions
3.1. Performance of RCC piles with helical grooves subjected to axial load
Pile performance under axial load is evaluated based on the pile’s load-settlement behaviour. In granular soils, such as sand, the pile’s performance is primarily influenced by the frictional resistance between the pile and soil, which is dependent on the pile surface area and soil properties. The numerical simulations were performed using the finite element method (FEM).
In , it’s evident that the control pile, when subjected to axial load, primarily relies on end bearing as the dominant load-resisting mechanism. This is because the control pile’s surface resistance is considerably lower than the end bearing. This is observed by the pressure bulb’s appearance as depicted in .
Figure 14. (a) Control pile subjected to axial load showing displacement of soil particles and (b) end bearing as pressure bulb.

In , it is observed that the RCC pile with a 100 mm pitch helical groove, under axial load, accumulates sand particles on the sides of the pile, especially in the region of the helical grooves. At each groove, the accumulated sand resists the downward movement of the pile and contributes to an increase in load-carrying capacity, as shown in .
Figure 15. (a) P100 helical groove pile subjected to axial load showing displacement of soil particles and (b) end bearing pressure and pressure at groove interlocks.

illustrates that the RCC pile with a 75 mm groove, under axial load, exhibits lower settlement compared to the RCC pile with 100 mm pitch helical grooves. In , lower pressure in the surrounding soil is observed. The settlement and pressure decrease as the number of helical grooves increases, owing to the interaction of the grooves with the surrounding soil.
Figure 16. (a) P75 helical groove pile subjected to axial load showing displacement of soil particles and (b) end bearing pressure and pressure at groove interlocks.

, it’s clear that the settlement is comparably lower than that of the RCC pile with 75 mm helical grooves. This is due to the presence of a higher number of helical grooves interacting with the soil. Consequently, both the pile’s settlement and the soil pressure decrease as shown in .
Figure 17. (a) P50 helical groove pile subjected to axial load showing displacement of soil particles and (b) end bearing pressure and pressure at groove interlocks.

In , the axial force within the pile is illustrated along its depth. For the control pile, it’s observed that the axial force decreases as the depth of the pile increases. This decrease in axial force is primarily due to the mobilization of axial force as skin friction. As the depth of the pile increases, the axial force in the pile decreases, reflecting the transition of axial force into skin friction. For the pile with helical grooves, a similar pattern is observed, with the axial force decreasing as the depth of the pile increases, much like the control pile. However, in the pile with helical grooves, the mobilization of forces occurs not only through skin friction but also through the interlocking of the grooves. As a result, the axial force in the pile becomes negligible within a relatively short depth of the pile. It’s also noted that as the pitch of the helical groove decreases, the depth at which the mobilization of forces occurs also decreases. It is also noted that the 100 mm depth of the pile is taken as a free board which means soil starts at 100 mm from the top of the pile. The axial forces are determined based on the average axial stress at different levels within the pile, multiplied by the cross-sectional area of the pile.
displays load settlement curves that are used for the assessment of the performance of RCC piles with helical grooves. The curves illustrate the settlement of the piles with incremental load increases for the applied loads. The P50 helical pile exhibits superior performance as compared to the P75, P100, and control piles. Specifically, the P50 helical pile can bear a 158% higher load than the control pile at the 10% diameter settlement criteria. Similarly, the P75 and P100 RCC piles with helical grooves bear 75 and 35% higher loads than the control pile, respectively in line with the experimental results. The load settlement curves are non-linear, and all the pile curves exhibit a similar type of non-linearity. The presence of helical grooves in the pile leads to an increase in the load-carrying capacity.
The numerical simulation results exhibit lower load resistance to the applied load on the application of loads, however, after the 4 mm settlement of the pile, the resistance offered is higher than the experimental results. However, the experimental and numerical load settlement curves exhibit a similar trend of non-linearity. At 10% of diameter criteria (8 mm settlement) the numerical results yield 8% to 10% higher settlement than the experimental results for all the different pitch variations.
The number of grooves increases as the pitch of the helix decreases, allowing for a greater volume of cohesionless material to interlock with the pile as shown in . The load-carrying capacity is dependent on the pitch of the helix, with a lower pitch resulting in a higher number of helices, and subsequently, an increase in the load-carrying capacity.
Table 4. The percentage volume of groove for a pitch of the helix.
quantifies both the end bearing and shaft resistance, which were determined by modelling the soil beneath the pile for end bearing and the soil surrounding the pile for shaft resistance. reveals that as the pitch of the grooves decreases, resulting in a greater number of grooves on the pile, the shaft resistance of the pile is significantly higher when compared to a plain pile. This indicates that the presence of helical grooves enhances the pile’s ability to resist axial loads, particularly in the shaft region. All the models are simulated for an axial load of 4 kN.
Table 5. End bearing and shaft resistance.
3.2. Performance of RCC piles with helical grooves subjected to lateral load
The piles are tested for lateral loads noting the lateral displacements for each incremental load. The load-displacement curves are used to represent the lateral soil resistance, which is a function of the soil stiffness and the lateral displacement of the pile. Moreover, the RCC pile with helical grooves system exhibited good resistance to lateral loads due to the presence of helical grooves, which provided additional lateral resistance. shows that the soil is in passive earth pressure, and the total lateral load mobilizes by passive earth pressure, a similar behaviour is also observed in the control pile.
depicts that the lateral displacement of the control pile is greater than that of the pile with helical grooves on the passive earth pressure side. On the active earth pressure side, the lateral displacement in the helical groove pile is higher than in the control pile. It’s noteworthy that for the pile with helical grooves, variations in the pitch of the grooves do not lead to significant changes in the lateral displacement of the pile throughout its depth.
The load-displacement curves of RCC piles with helical grooves are shown in . The lateral load resistance of helical piles increases as the pitch decreases. RCC pile with helical groove P50 pile resists 13.26%, similarly, P75 is 6.25% and P100 is 3% higher than the control pile at 12 mm and 5 mm displacement criteria as per experimental data shown in . Moreover, shows a good agreement between the experimental and numerical results. The experimental results show higher variation in lateral load resistance as the pitch of the helical groove decreases. However, the numerical results show a marginal difference in the lateral load resistance as the pitch of the helix decreases.
(1)
(1)
(2)
(2)
(3)
(3)
Where, Pu = ultimate lateral load
e = eccentricity of load above sand level
kp = passive earth pressure coefficient
ϕ = angle of internal friction
kp = 3.68 and Pu = 570 N
Safe load = 570/2.5 Factor of safety = 2.5
Safe load = 228 N.
In , a schematic diagram of lateral loading is presented. The safe lateral load for the control pile is calculated using EquationEquation 1(1)
(1) as per Broom’s method, resulting in a value of 228 N. It’s observed in that at a 5 mm lateral displacement, the lateral load is 278 N, and corresponding to a 12 mm lateral displacement, the calculated safe lateral load is 50% of 450 N, which is equal to 225 N. As per the relevant code (IS 2911 (Part 4), Citation2013), the safe lateral load is considered the lowest of the two conditions. Therefore, the safe lateral load for the control pile is determined to be 225 N. This finding indicates a good agreement between the experimental and theoretical results.
4. Conclusions
In this study, RCC piles with helical grooves and plain RCC piles are examined for axial and lateral loads by using experimental and numerical approaches. Based on the study the following conclusions are drawn.
Reinforced Concrete (RCC) piles with helical grooves exhibit enhanced performance in both axial and lateral load scenarios when compared to the control pile.
As the pitch of the helical groove decreases, the pressure within the surrounding soil also decreases. This reduction in pressure is attributed to the increased number of helical grooves, which play a role in mobilizing the applied axial load.
Axial force in the pile decreases along the depth of the pile as the pitch of the helical groove decreases.
RCC piles with a helical groove pitch of 50 mm demonstrated a remarkable 158% increase in axial load-carrying capacity compared to the control pile when analysed at a 10% settlement criterion. Similarly, piles with a pitch of 75 and 100 mm exhibit 75 and 35% higher axial load-carrying capacity, respectively, when compared to the control pile at the same settlement criterion.
RCC piles with a helical groove pitch of 50 mm offer 13.26% greater lateral load resistance compared to the control pile when assessed at both 5 and 12 mm displacement criteria. Similarly, piles with pitch sizes of 75 and 100 mm provide 6.25 and 3% higher lateral load resistance, respectively, compared to the control pile at these displacement criteria.
The inclusion of helical grooves in the pile enables the surrounding soil to interlock with the pile, effectively mimicking an end-bearing behaviour. This interaction leads to a substantial increase in the pile’s load-carrying capacity.
Disclosure statement
No potential conflict of interest was reported by the author(s).
Additional information
Notes on contributors
Arun Kumar Y. M.
Arun Kumar Y. M. received his Master of Technology in Structural Engineering from National Institute of Technology, Surathkal, Karnataka, India. Presently he is working as an Assistant Professor in the department of Civil Engineering, Manipal Institute of Technology, Manipal Academy of Higher Education, Manipal, India. His research interests include concrete technology and Soil-structure interaction. He has published several papers in reputed journals and conferences.

Kiran K. Shetty
Kiran K. Shetty received his doctoral degree form Manipal Academy of Higher Education (MAHE) Manipal, Karnataka, India. Presently he is working as a professor in the department of Civil Engineering, Manipal Institute of Technology, Manipal Academy of Higher Education, Manipal, India. He has more than 23 years of teaching and research experience. His current areas of research include Geopolymer Concrete, Alkali activated concrete, Artificial Aggregates, Structural Dynamics, soil-structure interaction and Earthquake Engineering. Currently he is guiding 6 PhD students. He has published several papers in reputed journals and conference proceedings.
A. Krishnamoorthy
A. Krishnamoorthy received his doctoral degree form Karnataka Regional Engineering College (KREC) Surathkal, Karnataka, India, presently known as National Institute of Technology, Surathkal, Karnataka, India. Presently he is working as a professor in the department of Civil Engineering, Manipal Institute of Technology, Manipal Academy of Higher Education, Manipal, India. He has more than 38 years of teaching and research experience. His current areas of research include soil-structure interaction, fluid-structure interaction, ground improvement techniques, isolation control techniques for structures. He has guided 5 PhD students. He has published several papers in reputed journals and conference proceedings.
References
- Abbasa, J. M., Chik, Z., & Taha, M. R. (2015). Influence of axial load on the lateral pile groups response in cohesionless and cohesive soil. Frontiers of Structural and Civil Engineering, 9(2), 176–193. https://doi.org/10.1007/s11709-015-0289-7
- Abusharar, S. W., Zheng, J. J., & Chen, B. G. (2009). Finite element modeling of the consolidation behavior of multi-column supported road embankment. Computers and Geotechnics, 36(4), 676–685. https://doi.org/10.1016/j.compgeo.2008.09.006
- Ali, H., Sarmad, A., & Wisam, S. (2016). Analysis of laterally loaded 2 × 2 square pile groups using finite element method. In 6th International Conference On Advances in Civil and Structural Engineering – CSE’16, December, 2017 (pp. 7–12). https://doi.org/10.15224/978-1-63248-110-8-27
- Bao, X., Wu, S., Liu, Z., Su, D., & Chen, X. (2022). Study on the nonlinear behavior of soil–pile interaction in liquefiable soil using 3D numerical method. Ocean Engineering, 258(July), 111807. https://doi.org/10.1016/j.oceaneng.2022.111807
- Baqi, Y., Khan, J. A., Ahmad, S., & Sadique, M. R. (2022). Numerical analysis of hollow steel pile subjected to a combination of horizontal and vertical loads. Materials Today: Proceedings, 65, 609–614. https://doi.org/10.1016/j.matpr.2022.03.195
- Chen, Y., Deng, A., Lu, F., & Sun, H. (2020). Failure mechanism and bearing capacity of vertically loaded pile with partially-screwed shaft: Experiment and simulations. Computers and Geotechnics, 118, 103337. https://doi.org/10.1016/j.compgeo.2019.103337
- Chen, Y., Deng, A., Wang, A., & Sun, H. (2018). Performance of screw–shaft pile in sand: Model test and DEM simulation. Computers and Geotechnics, 104, 118–130. https://doi.org/10.1016/j.compgeo.2018.08.013
- Chen, Y., Lv, Y., Wu, K., & Huang, X. (2022). Numerical analysis of bridge piers under earthquakes considering pile–soil interactions and water–pier interactions. Ocean Engineering, 266(P4), 113023. https://doi.org/10.1016/j.oceaneng.2022.113023
- Chiou, J. S., & Wei, W. T. (2021). Numerical investigation of pile-head load effects on the negative skin friction development of a single pile in consolidating ground. Acta Geotechnica, 16(6), 1867–1878. https://doi.org/10.1007/s11440-020-01134-0
- Choi, Y., Kim, D.-C., Kim, S.-S., Nam, M. S., & Kim, T.-H. (2013). Implementation of noise-free and vibration-free PHC screw piles on the basis of full-scale tests. Journal of Construction Engineering and Management, 139(8), 960–967. https://doi.org/10.1061/(asce)co.1943-7862.0000667
- Choo, J. (2018). Mohr–Coulomb plasticity for sands incorporating density effects without parameter calibration. International Journal for Numerical and Analytical Methods in Geomechanics, 42(18), 2193–2206. https://doi.org/10.1002/nag.2851
- Da Silva, B. O., Tsuha, C. D. H. C., & Dos Santos Filho, J. M. S. M. (2023). A database of installation monitoring and uplift load tests of round-shaft helical anchors in Brazil. Probabilistic Engineering Mechanics, 71, 103378. https://doi.org/10.1016/j.probengmech.2022.103378
- Dehestani, M., & Mousavi, S. S. (2015). Modified steel bar model incorporating bond-slip effects for embedded element method. Construction and Building Materials, 81, 284–290. https://doi.org/10.1016/j.conbuildmat.2015.02.027
- George, B. E., Banerjee, S., & Gandhi, S. R. (2020). Numerical analysis of helical piles in cohesionless soil. International Journal of Geotechnical Engineering, 14(4), 361–375. https://doi.org/10.1080/19386362.2017.1419912
- Geotechnica, S., & Do, K. (2016). The Non-Linear Mohr – Coulomb Model for Sands. January 2006.
- Hassan, S. A., Al-Soud, M. S., & Mohammed, S. A. (2019). Behavior of pyramidal shell foundations on reinforced sandy soil. Geotechnical and Geological Engineering, 37(4), 2437–2452. https://doi.org/10.1007/s10706-018-00767-z
- IS 2386-Part III (1963). Method of test for aggregate for concrete. Part III-Specific gravity, density, voids, absorption and bulking. Bureau of Indian Standards, New Delhi (Reaffirmed 2002).
- IS 2720: Part 13 (1986). Methods of test for soils, Part 13: Direct shear test. Bureau of Indian Standards, New Delhi, India. Reaffirmed (2002), 1–12.
- IS 2720: Part 14 (1983). Methods of test for soils: determination of density index (relative density) of cohesionless soils. Bureau of Indian Standards, New Delhi, India. Reaffirmed (2006), 1–14.
- IS 2720: Part 4 (1985). Indian standard, methods of test for soils, Part 4: Grain size analysis. Bureau of Indian Standards, New Delhi, India. Reaffirmed (2006), 1–38.
- IS 2911 (Part 4) (2013). Design and construction of pile foundations – Code of practice part 4 load test on piles. Bureau of Indian Standards, 2911 (December).
- IS:2911 (Part 1/Section 2) (2010). Design and construction of pile foundations – Code of practice. 2911(Part 4), 2911.
- Jawad, S., & Han, J. (2021). Numerical analysis of laterally loaded single free-headed piles within mechanically stabilized earth walls. International Journal of Geomechanics, 21(5), 1–14. https://doi.org/10.1061/(asce)gm.1943-5622.0001989
- Khodair, Y., & Abdel-Mohti, A. (2014). Numerical analysis of pile–soil interaction under axial and lateral loads. International Journal of Concrete Structures and Materials, 8(3), 239–249. https://doi.org/10.1007/s40069-014-0075-2
- Li, L., Liu, X., Liu, H., Wu, W., Lehane, B. M., Jiang, G., & Xu, M. (2022). Experimental and numerical study on the static lateral performance of monopile and hybrid pile foundation. Ocean Engineering, 255, 111461. https://doi.org/10.1016/j.oceaneng.2022.111461
- Liu, P., Liu, C., Zhang, S., Wang, Y., & Wang, Q. (2022). Depth-varying corrosion characteristics and stability bearing capacity of steel pipe piles under aggressive marine environment. Ocean Engineering, 266(P1), 112649. https://doi.org/10.1016/j.oceaneng.2022.112649
- Lu, J., Guang, H., Cui, L., Liu, J., Wang, C., & Kumar, S. A. (2023). Experimental study on penetration characteristics of an open-ended pile under static and dynamic driving methods. Soil Dynamics and Earthquake Engineering, 166, 107770. https://doi.org/10.1016/j.soildyn.2023.107770
- Mardfekri, M., Gardoni, P., & Roesset, J. M. (2013). Modeling laterally loaded single piles accounting for nonlinear soil-pile interactions. Journal of Engineering, 2013, 1–7. https://doi.org/10.1155/2013/243179
- Mittal, S., Ganjoo, B., & Shekhar, S. (2010). Static equilibrium of screw anchor pile under lateral load in sands. Geotechnical and Geological Engineering, 28(5), 717–725. https://doi.org/10.1007/s10706-010-9342-4
- Nowkandeh, M. J., & Choobbasti, A. J. (2021). Numerical study of single helical piles and helical pile groups under compressive loading in cohesive and cohesionless soils. Bulletin of Engineering Geology and the Environment, 80(5), 4001–4023. https://doi.org/10.1007/s10064-021-02158-w
- Ooi, P. S. K., Chang, B. K. F., & Wang, S. (2004). And pile groups. ASCE, 130, 1140–1151. https://doi.org/10.1061/(ASCE)1090-0241(2004)130
- Poulos, H. G. (2018). Pile load testing. Tall building foundation design (pp. 389–416). CRC Press. https://doi.org/10.1201/9781315156071-13
- Rao, N., & Prasad, Y. v s n (1993). Estimation of uplift capacity of helical anchors in clays. Journal of Geotechnical Engineering, 119(2), 352–357. https://doi.org/10.1061/(ASCE)0733-9410(1993)119:2(352)
- Saha, R., Dutta, S. C., Haldar, S., & Kumar, S. (2020). Effect of soil-pile raft-structure interaction on elastic and inelastic seismic behaviour. Structures, 26, 378–395. https://doi.org/10.1016/j.istruc.2020.04.022
- Shen, W., Pang, Q., Fan, L., Li, P., & Zhao, X. (2023). Monitoring and quantification of non-uniform corrosion induced mass loss of steel piles with distributed optical fiber sensors. Automation in Construction, 148, 104769. https://doi.org/10.1016/j.autcon.2023.104769
- Soltani-Jigheh, H., & Zahedi, P. (2020). Load transfer mechanism of screw piles in sandy soils. Indian Geotechnical Journal, 50(6), 871–879. https://doi.org/10.1007/s40098-020-00431-5
- Srijaroen, C., Hoy, M., Horpibulsuk, S., Rachan, R., & Arulrajah, A. (2021). Soil–cement screw pile: Alternative pile for low- and medium-rise buildings in soft Bangkok clay. Journal of Construction Engineering and Management, 147(2), 04020173. https://doi.org/10.1061/(asce)co.1943-7862.0001988
- Taborda, D. M. G., Pedro, A. M. G., & Pirrone, A. I. (2022). A state parameter-dependent constitutive model for sands based on the Mohr-Coulomb failure criterion. Computers and Geotechnics, 148, 104811. https://doi.org/10.1016/j.compgeo.2022.104811
- Venkatesan, V., & Mayakrishnan, M. (2022). Behavior of mono helical pile foundation in clays under combined uplift and lateral loading conditions. Applied Sciences, 12(14), 6827. https://doi.org/10.3390/app12146827
- Vignesh, V., & Muthukumar, M. (2023). Experimental and numerical study of group effect on the behavior of helical piles in soft clays under uplift and lateral loading. Ocean Engineering, 268, 113500. https://doi.org/10.1016/j.oceaneng.2022.113500
- Yang, Y., Ouyang, W., Liu, K., & Liu, S. W. (2022). Efficient numerical algorithms for assessing the mechanical performance of corroded offshore steel sheet piles. Ocean Engineering, 266(P2), 112776. https://doi.org/10.1016/j.oceaneng.2022.112776
- Zarrabi, M., & Eslami, A. (2016). Behavior of piles under different installation effects by physical modeling. International Journal of Geomechanics, 16(5), 1–12. https://doi.org/10.1061/(asce)gm.1943-5622.0000643
- Zhang, Q., & Zhuo, W. (2014). Numerical analysis of the mechanical properties of batter piles under inclined loads. Journal of Highway and Transportation Research and Development, 8(2), 66–71. https://doi.org/10.1061/JHTRCQ.0000383
- Zheng, G., Wang, R., Lei, H., Zhang, T., & Fan, Q. (2023). Load-transfer-associated settlements of a piled building during shield tunnelling in soft ground. Tunnelling and Underground Space Technology, 133, 104964. https://doi.org/10.1016/j.tust.2022.104964
- Zhou, S., Zhou, M., Tian, Y., & Zhang, X. (2023). Effects of strain rate and strain softening on the installation of helical pile in soft clay. Ocean Engineering, 285(P1), 115370. https://doi.org/10.1016/j.oceaneng.2023.115370