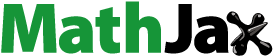
Abstract
Optimizing the efficiency of metal powder production through brush atomization necessitates a comprehensive understanding of the interaction between the brush bristles and the molten metal, as well as its consequential effects on the fragmentation of the melt. Thus this study presents Computational Fluid Dynamics (CFD) models of the brush-melt interplay during the atomization of molten metals. The volume-of-fluid method was used to examine the various stages of melt fragmentation, from when the melt is discharged to when it settles on the brush bristles. The results revealed that the first breakup occurred close to the discharge orifice due to aerodynamic instability and fell within 13 < < 40.3. The nature of this breakup depends on the brush speed, bristle length, bristle diameter, brush density, and melt properties. A second breakup was observed when the melt contacted the rotating bristles. The melt bridges formed between two or more bristles due to attractive forces between the closely packed bristles induced by the capillary force of the melt and the surface tension of the meniscus surface are also overcome due to brush rotation. These results are in agreement with the experimental observations and are therefore sufficient for demonstrating the system.
1. Introduction
The application of metal powders in advanced powder metallurgy processes requires high-quality powders with very small particle sizes. Poor-quality metal powders can result in various issues or imperfections in final products. These issues include pores, cracks, inclusions, and residual stresses (Gibbons et al., Citation2023; Kassym & Perveen, Citation2020; Whiting et al., Citation2019). Although many prevailing metal atomization technologies are sufficient to produce powders for both existing and emerging applications, they are always at the expense of system complexity, high energy, and cost (Phairote et al., Citation2012; Sungkhaphaitoon et al., Citation2017; Xie et al., Citation2004; Zhao, Citation2006). The novel brush atomizer shown in was developed by Onwuka et al. (Citation2021) as a solution to these drawbacks.
This technology offers a simple, energy-friendly, economical, and low-cost solution for producing micro-sized metal powders. This machine comprises two basic units: a furnace and an atomization chamber. The atomization unit uses brushes with sturdy bristles to produce the metal powders. Fine metal spatters are produced when the wetted rotating brush bristles carrying the melt film are flicked upon contact with obstacles. These spatters solidify into powder upon cooling.
To enhance the powder quality of this machine and manipulate the system yield, it is essential to understand the complex process of bristle-melt interaction and how it influences melt fragmentation. Thus, employing procedures that permit easy tweaking of process parameters at a minimal cost could be beneficial. Studies conducted by Zeoli et al. (Citation2008), Sarkar et al. (Citation2016), and Kayali and Unal (Citation2018) utilized Computational Fluid Dynamics (CFD) modeling as an effective approach to understanding the underlying thermal physics of powder atomization.
To accurately represent the atomization process through CFD simulations, it is essential to effectively capture complex deforming interfaces, which can be achieved using the volume of fluid (VOF) method, a numerical technique designed for flows with sharp moving and deforming interfaces (Hirt & Nichols, Citation1981; Katopodes, Citation2019). Utilizing the VOF approach allows for a comprehensive depiction of pertinent details pertaining to the disintegration of liquid sheets. This includes critical elements such as the precise location of perforation and droplet formation, as well as the mechanism by which the sheet breaks. Additionally, it yields reasonably accurate predictions regarding the primary droplet formation and velocity at moderate to high melt mass flow rates (Li & Fritsching, Citation2017). However, the VOF method exhibits inaccuracies in computing critical factors such as unit normal vector, mean curvature, and surface tension force (Ningegowda & Premachandran, Citation2014). ANSYS Fluent, which is recognized for its exceptional CFD capabilities, is one of the most powerful software options for conducting VOF studies (Heng Yeoh & Barber, Citation2009; Lorstad & Fuchs, Citation2001; Paz et al., Citation2019). In a study conducted by Zeoli et al. (Citation2011), complex fluids were examined during atomization from various nozzle designs using the VOF method and the Reynolds Stress Model (RSM). The flow equations were solved using a pressure-based solver with second-order implicit time discretization. The findings from this study indicate that the gas swirl atomizer appears to hinder the atomization process by stabilizing the melt core. Conversely, the inner jet atomizer has the potential to reduce the amount of melt material traveling along the centerline of the atomization chamber, thereby enhancing powder production. Wu et al. (Citation2017) utilized a multiphase axisymmetric CFD domain to explore the effects of varying the operating conditions on the interaction between a liquid metal stream and a water jet. To simulate this phenomenon, the researchers employed the VOF model coupled with the standard k-ω turbulence model. The results of their investigation demonstrated that increasing the apex angle led to increased energy transfer into the metal stream, consequently resulting in the production of smaller droplets in the primary atomization zone. Ghiji et al. (Citation2014) employed Large Eddy Simulation (LES) to model the primary atomization occurring in a high-pressure diesel jet. The Eulerian framework was utilized by employing the volume of fluid phase fraction-based interface capturing technique. The obtained results highlight an increased jet breakup with higher mesh resolutions. Additionally, the investigation of the in-nozzle flow indicated a non-axisymmetric behavior. To explore the influence of the liquid mass feeding rate, disc spinning speed, disc radius, liquid viscosity, density, and surface tension on the liquid film thickness at the disc edge, Pan et al. (Citation2014) developed a steady-state, two-dimensional, and multiphase CFD model. Through parametric numerical experiments, they discovered that the disc radius and spinning speed, as well as the liquid mass feeding rate, viscosity, and density, had a substantial impact on the liquid film thickness. In contrast, the liquid surface tension exhibited negligible effects. Additionally, the simulated results were compared with the experimental data, and satisfactory agreement was observed. Motaman (Citation2013) examined the behavior of single-phase gas flow during close-coupled gas atomization using optical Schlieren and numerical CFD techniques. Analysis of the CFD results indicated that no flow separation occurred around the external wall of the melt tip for any of the nozzles investigated at a gas pressure of 0.5 MPa. Moreover, the numerical model, along with the tested boundary conditions, was in good agreement with the experimental tests, thereby providing successful numerical validation. There have been no studies to understand the melt breakup process in brush atomization. Understanding the factors influencing melt fragmentation, such as brush speed, bristle length, diameter, and density, allows for more precise control over the production of metal powders. This is crucial for ensuring that the resulting powders meet specific requirements, such as controlled particle sizes, which is essential for various industrial applications. Thus the VOF interface capturing technique was employed in this research to examine the melt behavior from the point of leaving the discharge orifice of the furnace up to where it makes contact with the brush bristles. Further, the melt breakup that occurs when the brush bristles are flicked by the obstacles will be discussed in a future study.
2. Methodology
2.1. Computational fluid dynamics modeling
2.1.1. Assumptions
The melt film flow was assumed to be incompressible, Newtonian and isothermal.
2.1.2. Governing equations
The continuity, mass, momentum, and energy equations are given by EquationEquations (1)–(4) (Alibek et al., Citation2018; Avijit & Sumanta, Citation2021; Gao et al., Citation2003; Ketabdari, Citation2016):
Continuity Equation:
(1)
(1)
Momentum Equation:
(2)
(2)
Mass Equation:
(3)
(3)
Energy Equation:
(4)
(4)
Where and
are energy and temperature respectively.
The density and viscosity of the phases are given by EquationEquations (5)(5)
(5) and Equation(6)
(6)
(6) respectively:
(5)
(5)
(6)
(6)
Where and
are the liquid and gas phases respectively.
Volume Fraction:
(7)
(7)
The effects of surface tension along the interface between each pair of phases were modeled using the Continuum Surface Stress (CSS) method. The surface stress tensor due to the surface tension is represented by EquationEquation (8)(8)
(8) (Roshdi et al., Citation2018):
(8)
(8)
(9)
(9)
(10)
(10)
(11)
(11)
where
= unit tensor,
= surface tension coefficient,
= tensor product of the two vectors: the original normal and the transformed normal,
= volume fraction,
= volume fraction gradient.
If is the contact angle at the wall, then the surface normal at the live cell next to the wall is given by EquationEquation (12)
(12)
(12) (Malgarinos et al., Citation2014)
(12)
(12)
where
and
are the respective unit vectors that are normal and tangential to the wall.
2.1.3. Model implementation
The models were implemented in 2D geometry, as shown in . was generated using Autodesk Inventor 2016 and discretized in Fluent mesh. To precisely capture detailed information on the melt disintegration around the brush bristles, a finer mesh was applied at the melt inlet and bristles than in other areas. The boundary conditions for this domain are shown in .
A transient pressure-based solver was used with the VOF multiphase model coupled with energy models to account for the heat transfer in the process. The primary and secondary phases were set as air and aluminum, respectively, whereas the bristles as mild steel walls. The domain was set to be filled with air, and the volume fraction of the aluminum melt at the entrance (velocity inlet) was set to 1.0. The wall contact angle, which is used to adjust the surface normal in the cells near the wall, was specified as 130° (Mafoud, Citation1992). The effect of surface tension was modeled using the Continuum Surface Stress (CSS) model, whereas the k-ɛ model was used to account for turbulence. A dynamic mesh with smoothing and remeshing, as well as the user-defined function (UDF) shown in Appendix A (i), was used to achieve brush rotation. The properties of air and aluminum used in this analysis are listed in . The models were solved using an implicit finite-volume method, and second-order spatial and temporal discretization schemes were employed. This solution procedure uses the Pressure Implicit with Split Operator (PISO) algorithm in conjunction with conjugate gradient methods. The UDF shown in Appendix A (ii) was used to evaluate the droplet statistics, that is, the number of droplets and droplet diameter after each simulation. The effects of brush diameter number of bristles
brush speed,
and melt velocity,
on melt disintegration were also investigated. To ensure that the simulation results were robust and independent of mesh size, a mesh independence test was performed. Six meshes with total grid elements of 17,338, 56,348, 193,249, 604,859, 1,538,127 and 1,743,513, were simulated under the same conditions until convergence (1e-4). It was observed that beyond 1,538,127 elements, further refinement did not significantly affect solution accuracy. Therefore, a mesh of 1,538,127 elements was used in this study.
Table 1. Properties of the physical variables.
2.2. High speed imaging of the melt-bristle interaction and drop formation process
The equipment used for this study was a Nikon camera, camera timer, external flash, 100 mm macro lens, black background, light reflector (aluminum foil), tripod, and strobe light. Aluminum was used as the test material in this study. The camera was mounted on a tripod and placed 150 cm from the brush bristles, with the reflector and strobe light placed on the sides of the machine. With the camera set on manual focus, its focus was adjusted to the melt-bristle interface. In addition, the ISO, shutter speed, and aperture were varied between 200–400, 1/250s–1/1000s and f/4–f/8 respectively. With the timer set at 8s and the brush with bristle diameter 0.03 mm operated at 2000 rpm, the room light was turned off, and on expiration for 8s, images were captured and viewed. The obstacles were removed such that the brush rotated without deflecting the bristles. The room light was turned on, and the machine was turned off. This process was repeated with the ISO, aperture, and shutter speeds adjusted until the required number of shots was obtained. The analysis of particle size was evaluated using Image Pro Plus software.
3. Results and discussion
3.1. Airflow analysis of the rotating brush
The velocity vector of the brush with bristle diameter, melt velocity
number of bristles
= 140, and brush speed
= 2000 rpm are presented in . The airflow velocity due to brush rotation increased from the center to the tip of the bristles, reaching its maximum, and then decreased as it moved outward. In this region, the airflow displayed disorganized patterns because it was not guided. The aerodynamic force due to this flow circulation first acts on the melt before it makes contact with the brush bristles. The nature of the breakup experienced in this regime falls within the second wind-induced region in the jet stability curve (Ketabdari, Citation2016), which is 13 <
< 40.3.
The effects of brush speed, bristle diameter, and number of bristles on air velocity are shown in . Generally, the air velocity increased as the brush accelerated from rest and decreased to a steady value. However, with the brush speed, at 2000 rpm, 4220 rpm, 5000 rpm, 10,500 rpm and 14,500 rpm while the other parameters were kept constant at
and
the velocity plot in reveals an increase in velocity with increasing brush speed. This trend was also consistent for other bristle diameters, numbers of bristles, and melt velocities when the speed was varied. Also, for a constant
and
and varying bristle diameter,
= 0.01 mm, 0.03 mm and 0.06 mm, the airflow velocity increased with increasing bristle diameter as shown in (b). The number of bristles,
also affects the airflow velocity, as shown in . With
2000 rpm, and
remained constant, and
varied at 60, 100, 140, and 200 bristles; the air velocity increased with an increasing number of bristles.
3.2. Analysis of the melt-brush interaction
The volume of fluid (VOF) capture of the location of the melt perforation and droplet formation at different times is shown in . shows the melt when it left the orifice. The melt was distorted in the airflow direction owing to the short jet length and minimal air impact.
As the melt continued to grow (), the jet became more unstable, particularly as it approached the zone of maximum airflow. Aerodynamic forces cause greater instability around the surface of the melt, which fragments the melt film. This represents the first breakup observed during atomization. shows how different child droplets from the first breakup adhere and move with the brush. The sizes of the child droplets produced for different simulated parameters were between 564 µm and 8 mm. Note that this process occurs before the bristles are flicked by obstacles, where further breakup is expected.
shows the VOF contours of brushes with 60, 100, 140, and 200 bristles. Apart from the fact that airflow increases with increasing bristles, closely packed bristles tend to form melt bridges compared with loosely packed bristles. However, instabilities and adhesion cause smaller droplets to cling on each bristle when bridges are broken. These factors generally favor the production of narrow-sized particles. The size of these droplets depends on the number of bristles per unit of melt surface area. The droplet diameters for different numbers of bristles are shown in . A more significant number of narrow-sized droplets was produced when the bristles were closely packed. In addition, the number of droplets increases with an increase in the number of bristles.
The particle size distribution for brushes with brush diameter 0.01 mm, 0.03 mm and 0.06 mm are presented in . A decrease in the number of droplets was observed as the bristle diameter increased. Although a larger bristle diameter suggests a greater surface area for the melt to adhere to, leading to larger droplets, it is important to note that larger bristle diameters also generate stronger aerodynamic forces that can rupture the melt film.
The particle size distributions for brush speeds of 2000 rpm, 4220 rpm, 5000 rpm and 10,500 rpm are shown in . It can be observed that the number of droplets on the bristles decreases with increasing speed. As observed earlier, the higher the brush speed, the greater the airflow velocity, and consequently, the greater the circulation. However, at very high speeds, the velocity of the airflow around the rotating brush is strong enough to blow the melt away from the bristles, resulting in a lower yield.
3.4. Analyses of high speed images
show the images captured at different instances during the high-speed imaging study. A melt film breakup was first observed close to the tip of the discharge orifice (). This breakup is the result of instabilities created by the aerodynamic forces.
As the melt flows out through the discharge orifice of the furnace, aerodynamic forces due to the swirling air generated as the brush rotates create instability against the effect of surface tension and rupture it into large ligaments with small droplets peeled off at the interface. The nature of the breakup depends on the melt flow properties and aerodynamic forces. When the metal started to flow out of the orifice, a gradual flow with a smaller jet diameter was observed. The nature of the breakup observed was chaotic, resulting in smaller child droplets that were rapidly thrown off the brush domain, preventing interaction with the rotating brush bristles, and thus further disintegration (). However, at its pour point, it was characterized by a higher flow rate and larger jet diameter, which resulted in larger ligaments with longer jet lengths before breakup (). Another breakup occurs when fragmented melts fall on brush bristles. Attractive forces between the bristles, induced by the capillary force of the melt and the surface tension of the meniscus surface, create melt bridges between two or more bristles. In other cases, droplets that are not large enough to form melt bridges form axisymmetric drops on the bristles. These resulting child droplets are approximately the size of the bristles in some cases and slightly larger than the bristle diameter in others. This agrees with the results obtained in the CFD study, which implies that the model is suitable for describing the system ().
Flow down the bristles is limited because of the viscosity of the melt and centrifugal forces, amongst other factors. It is greatly by Kapitza and centrifugal instabilities; hence, it is highly unstable and could even result in the destruction of the bridges created. A focused inquiry into how the melt behaves upon hitting the obstacles in the atomization chamber is essential to further advance and provide valuable insights into the final particle size and the subsequent solidification of the spattered particles.
4. Conclusion
This research sheds light on the complex dynamics involved in brush atomization and offers valuable insights into process optimization for the production of metal powders with controlled particle sizes. The key findings from this study include:
Melt fragmentation was first observed close to the melt discharge orifice, which occurred due to aerodynamic forces caused by brush rotation.
The nature of this initial breakup is influenced by various parameters, including brush speed, bristle length, bristle diameter, and brush density.
A second breakup was observed when the melt contacted the rotating brush bristles, forming melt bridges and smaller droplets. These findings are consistent with the experimental observations and validate the effectiveness of the computational model.
The utilization of closely packed bristles with a diameter of 0.01mm, operating at moderate speeds ranging from 2000 rpm to 4220 rpm, favored powders with a narrow size distribution and high yield.
Authors contribution
Onwuka O.S.: Conceptualization, methodology, writing the original draft. Unachukwu G.O.: Supervision, Writing – review and editing. Nwanya S.C.: Supervision, reviewing, methodology.
Nomenclature | ||
Roman letters | = | |
Symbols | = | Description |
g | = | Acceleration due to gravity [m/s2] |
keff | = | Effective thermal conductivity [J/kg] |
= | Number of time step [-] | |
= | Volume fraction gradient [-] | |
= | Surface normal at the live cell next to the wall [-] | |
= | Unit vectors normal and tangential to the wall respectively [-]. | |
= | Static pressure of fluid [N/m2] | |
= | The droplets radius [mm] | |
= | Time [s] | |
= | Velocity vector [m/s] | |
= | Energy [J] | |
= | External body forces [N] | |
= | Surface tension force [N] | |
= | Unit tensor [-] | |
= | Source Term | |
= | Surface stress tensor due to surface tension [-] | |
= | Temperature [°C] | |
= | Weber number of the gas phase [-] |
Greek letters | ||
Symbols | = | Description |
= | Volume fraction [-] | |
= | Surface tension [N/m] | |
= | Temperature of fluid [°C] | |
= | Velocity [m/s] | |
= | Viscosity [kg/m-s] | |
= | Density [kg/m3] | |
= | Wall contact angle [°] |
Disclosure statement
No potential conflict of interest was reported by the author(s).
Data availability statement
All data generated or analyzed during this study are included in this manuscript.
Additional information
Notes on contributors
Osinachi Stanley Onwuka
Osinachi Stanley Onwuka is a Lecturer at Michael Okpara University of Agriculture, Umudike, Nigeria. His research interests include: metal powder atomization, material synthesis, optimization and modeling of systems. Onwuka boasts a remarkable academic track record, featuring numerous publications and patented innovations.
Godwin Ogechi Unachukwu
Godwin Ogechi Unachukwu is a Professor in the field of Energy and Power at University of Nigeria, Nsukka. His expertise extends to areas such as energy efficiency, renewable energy, and energy policy. Professor Unachukwu is a beacon, inspiring the next generation of engineers and researchers.
Stephen Chijioke Nwanya
Stephen Chijioke Nwanya is a Professor at the University of Nigeria, Nsukka, whose contributions extend across education, research, and professional practice. He earned a Doctor of Philosophy Degree (Ph.D.) in Tecnologie Chimiche ed Energetiche at Universita’ Degli Studi di Udine, Italy. His interests include engineering management, energy modeling, and optimization.
References
- Alibek, I., Yeldos, Z., & Aida, N. (2018). Numerical simulation of dam break flow for various forms of the obstacle by VOF method. International Journal of Multiphase Flow, 109, 191–206. https://doi.org/10.1016/j.ijmultiphaseflow.2018.08.003
- ANSYS Inc. (2009). ANSYS FLUENT 12.0 UDF manual. ANSYS Inc.
- Avijit, K., & Sumanta, A. (2021). Numerical simulation of falling film flow hydrodynamics over round horizontal tubes. International Journal of Heat and Mass Transfer, 173, 121175.
- Eureka.im. (2020). FLUENT 6 - UDF to count the number of droplets in a VOF simulation. https://www.eureka.im/1249.html.
- Gao, D., Morley, N. B., & Dhir, V. (2003). Numerical simulation of wavy falling film flow using VOF method. Journal of Computational Physics, 192(2), 624–642. https://doi.org/10.1016/j.jcp.2003.07.013
- Ghiji, M., Goldsworthy, L., Garaniya, V., Brandner, P. A., & Hield, P. (2014). CFD modeling of primary atomization of diesel spray [Paper presentation]. 19th Australasian Fluid Mechanics Conference, Melbourne, Australia, 8–11 December.
- Gibbons, D. W., Govender, P., & van der Merwe, A. F. (2023). Metal powder feedstock evaluation and management for powder bed fusion: a review of literature, standards, and practical guidelines. Progress in Additive Manufacturing. https://doi.org/10.1007/s40964-023-00484-x
- Heng Yeoh, G., & Barber, T. (2009). Assessment of interface capturing methods in computational fluid dynamics (CFD) codes-A case study. The Journal of Computational Multiphase Flows, 1(2), 201–215. https://doi.org/10.1260/175748209789563946
- Hirt, C. W., & Nichols, B. D. (1981). Volume of fluid (VOF) method for the dynamics of free boundaries. Journal of Computational Physics, 39(1), 201–225. https://doi.org/10.1016/0021-9991(81)90145-5
- Kassym, K., & Perveen, A. (2020). Atomization processes of metal powders for 3D printing. Materials Today: Proceedings, 26(2), 1727–1733. https://doi.org/10.1016/j.matpr.2020.02.364
- Katopodes, N. D. (2019). Chapter 12 – Volume of fluid method. In N. D. Katopodes (Ed.), Free-surface flow (pp. 766–802). Butterworth-Heinemann. https://doi.org/10.1016/B978-0-12-815485-4.00018-8
- Kayali, Y. E., & Unal, R. (2018). Determination of metal powder particle size by numerical modeling in gas atomization. Journal of Faculty of Engineering and Architecture of Gazi University, 33(3), 1135–1144.
- Ketabdari, M. J. (2016). Free surface flow simulation using VOF method. In Numerical simulation – From brain imaging to turbulent flows. InTech.
- Li, X., & Fritsching, U. (2017). Process modeling pressure-swirl-gas-atomization for metal powder production. Journal of Materials Processing Technology, 239, 1–17. https://doi.org/10.1016/j.jmatprotec.2016.08.009
- Lorstad, D., & Fuchs, L. (2001). A volume of fluid (VOF) method for handling solid objects using fixed Cartesian grids. Transactions on Modeling and Simulation, 29, 143–152.
- Mafoud, M. (1992). The wettability of ceramic-coated steel substrates by liquid metals [M.Eng. thesis]. Faculty of Graduate Studies, Mining and Metallurgical Engineering, McGill University.
- Malgarinos, I., Nikolopoulos, N., Marengo, M., Antonini, C., & Gavaises, M. (2014). VOF simulations of the contact angle dynamics during the drop spreading: Standard models and a new wetting force model. Advances in Colloid and Interface Science, 212, 1–20. https://doi.org/10.1016/j.cis.2014.07.004
- Motaman, S. (2013). High-speed imaging and computational modeling of close-coupled gas atomization [Ph.D. thesis]. Institute for Materials Research, School of Process, Environmental and Materials Engineering, University of Leeds.
- Ningegowda, B. M., & Premachandran, B. (2014). A coupled level set and volume of fluid method with multi-directional advection algorithms for two-phase flows with and without phase change. International Journal of Heat and Mass Transfer, 79, 532–550. https://doi.org/10.1016/j.ijheatmasstransfer.2014.08.039
- Onwuka, O. S., Unachukwu, G. O., & Nwanya, S. C. (2021). Design and development of a brush atomization machine for metal powder production. Scientific African, 14, e00986. https://doi.org/10.1016/j.sciaf.2021.e00986
- Pan, Y., Witt, P. J., Kuan, B., & Xie, D. (2014). CFD Modeling of the Effects of Operating Parameters on the Spreading of Liquids on a Spinning Disc. The Journal of Computational Multiphase Flows, 6(1), 49–64. https://doi.org/10.1260/1757-482X.6.1.49
- Paz, C., Suárez, E., Vence, J., & Cabarcos, A. (2019). Analysis of the volume of fluid (VOF) method for the simulation of the mucus clearance process with CFD. Computer Methods in Biomechanics and Biomedical Engineering, 22(5), 547–566. https://doi.org/10.1080/10255842.2019.1569637
- Phairote, P., Plookphol, T., & Wisutmethangoon, S. (2012). Design and development of a centrifugal atomizer for producing zinc metal powder. International Journal of Applied Physics and Mathematics, 2(2), 77–82. https://doi.org/10.7763/IJAPM.2012.V2.58
- Roshdi, S., Kasiri, N., & Rahbar-Kelishami, A. (2018). VOF simulation of single rising drops in three liquid-liquid extraction systems using CSF and CSS interfacial force models. Brazilian Journal of Chemical Engineering, 35(4), 1315–1331. https://doi.org/10.1590/0104-6632.20180354s20170609
- Sarkar, S., Sivaprasad, P. V., & Bakshi, S. (2016). Numerical modeling and prediction of particle size distribution during gas atomization molten tin. Atomization and Sprays, 26(1), 23–51. https://doi.org/10.1615/AtomizSpr.2015011680
- Sungkhaphaitoon, P., Wisutmethangoon, S., & Plookphol, T. (2017). Influence of process parameters on zinc powder produced by centrifugal atomization. Materials Research, 20(3), 718–724. https://doi.org/10.1590/1980-5373-mr-2015-0674
- Whiting, J. G., Tondare, V. N., Scott, J. H. J., Phan, T. Q., & Donmez, M. A. (2019). Uncertainty of particle size measurements using dynamic image analysis. CIRP Annals, 68(1), 531–534. https://doi.org/10.1016/j.cirp.2019.04.075
- Wu, C., Asgarian, A., Chatterjee, S., & Paserin, V. (2017). Understanding water jet and metal stream interactions during water atomization of steel powders using analytical and CFD modeling techniques [Paper presentation]. International Conference on Powder Metallurgy and Particulate Materials (POWDERMET), Las Vegas, USA, June 13–16.
- Xie, J. W., Zhao, Y. Y., & Dunkley, J. J. (2004). Effects of processing conditions on powder particle size and morphology in centrifugal atomization of tin. Powder Metallurgy. 47(2), 168–172. https://doi.org/10.1179/003258904225015482
- Zeoli, N., Gu, S., & Kamnis, S. (2008). Numerical modeling of metal droplet cooling and solidification. International Journal of Heat and Mass Transfer, 51(15–16), 4121–4131. https://doi.org/10.1016/j.ijheatmasstransfer.2007.11.044
- Zeoli, N., Tabbara, H., & Gu, S. (2011). CFD modeling of primary break up during metal powder atomization. Chemical Engineering Science, 66(24), 6498–6504. https://doi.org/10.1016/j.ces.2011.09.014
- Zhao, Y. Y. (2006). Considerations in designing a centrifugal atomizer for metal powder production. Materials & Design, 27(9), 745–750. https://doi.org/10.1016/j.matdes.2005.01.011
Appendix A
(i) User Defined Function (UDF) for brush rotation (ANSYS Inc, Citation2009)
# include "udf.h"
DEFINE_CG_MOTION(rotation_udf_45, dt, vel, omega, time, dtime)
{
real pi = 3.141592;
real omega_constant = 2000*pi/180;
omega[2] = omega_constant;
}
(ii) UDF for counting the number of droplets in the VOF simulation (Eureka.im, Citation2020).
*/
#include "udf.h"
#define PHASE_INDEX 1
#define INTERFACE_VOF 0.5
#define NMAX 10000
#define MAX_DROPLET 1000000
DEFINE_ON_DEMAND
{
Domain *d;
Thread *t, *t0;
cell_tc, c0;
face_tf;
Thread *tf;
int i,n,n_child,N_search;
int N_droplet = 0;
cell_t cell_id1[NMAX], cell_id2[NMAX];
Thread *thread_id1[NMAX], *thread_id2[NMAX];
real Vol_droplet[MAX_DROPLET], Dia_droplet[MAX_DROPLET];
Thread**pt,**pt2;
d = Get_Domain(1);
/* initialization */
thread_loop_c(t,d)
{
begin_c_loop(c,t)
{
C_UDMI(c,t,0)=0;
}
end_c_loop(c,t)
}
/* droplet counting */
thread_loop_c(t,d)
{
pt = THREAD_SUB_THREADS(t);
begin_c_loop(c,t)
{
Vol_droplet[N_droplet] = 0.0;
if(C_VOF(c,pt[PHASE_INDEX])>INTERFACE_VOF && C_UDMI(c,t,0)==0)
{
C_UDMI(c,t,0)=N_droplet + 1;
Vol_droplet[N_droplet] + = C_VOLUME(c,t)*C_VOF(c,pt[PHASE_INDEX]);
N_search = 1;
cell_id1[0]=c;
thread_id1[0]=t;
do
{
n_child= 0;
for(i = 0; i < N_search; i++)
{
c_face_loop(cell_id1[i], thread_id1[i], n)
{
f = C_FACE(cell_id1[i], thread_id1[i], n);
tf = C_FACE_THREAD(cell_id1[i], thread_id1[i], n);
c0 = F_C0(f,tf);
t0 = THREAD_T0(tf);
if (c0==cell_id1[i] && THREAD_T1(tf) != NULL)
{
c0 = F_C1(f,tf);
t0 = THREAD_T1(tf);
}
pt2 = THREAD_SUB_THREADS(t0);
if(C_VOF(c0,pt2[PHASE_INDEX])>INTERFACE_VOF && C_UDMI(c0,t0,0)==0)
{
C_UDMI(c0,t0,0)=N_droplet + 1;
cell_id2[n_child]=c0;
thread_id2[n_child]=t0;
Vol_droplet[N_droplet] + = C_VOLUME(c0,t0)*C_VOF(c0,pt2[PHASE_INDEX]);
n_child + = 1;
if((n_child-1)==NMAX)
{
Message("Please increase NMAX! n");
exit(0);
}
}
}
}
N_search = n_child;
for(i = 0; i < NMAX; i++)
{
cell_id1[i]=cell_id2[i];
thread_id1[i]=thread_id2[i];
cell_id2[i] = 0;
thread_id2[i]=NULL;
}
}
while(N_search > 0);
N_droplet + = 1;
if((N_droplet-1)==MAX_DROPLET)
{
Message("Please increase MAX_DROPLET in the udf!! n");
exit(0);
}
}
}
end_c_loop(c,t)
}
Message("nn-------------------------------------n");
Message("Total number of droplets: %dn",N_droplet);
Message("-------------------------------------nn");
if(N_droplet!=0)Message(" ID DIAMETER(m) n");
for(i = 0; i < N_droplet; i++)
{
if(ND_ND==3)Dia_droplet[i] = 2.0*pow(0.75*Vol_droplet[i]/M_PI,0.333333333) ;
if(ND_ND==2)Dia_droplet[i] = 2.0*pow(Vol_droplet[i]/M_PI,0.5) ;
Message(" %d %gn",i + 1,Dia_droplet[i]);
}
}
/*