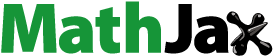
Abstract
Present work contains a 3-D finite element (FE) model created in Abaqus to examine bond behavior in controlled/non-corroded reinforced concrete beams. Study evaluated the compatibility between corrosion levels and beam deflections. Cohesive surface-based interaction method is applied to the Data obtained from recent studies in which corrosion was induced by accelerated corrosion technique. The cohesive surface-based interaction approach has been found to be suitable for simulating the interfacial binding between reinforcement bars and the concrete. Analysis of Control and corroded beams along with mesh sensitivity were used for the validation of the work. It is found that maintaining uniform mesh size for steel and concrete is necessary as varying mesh size influences the load – deflection relation. The developed FE model of RC beam under corrosion could be adopted to obtain the load at failure with considerable accuracy. Hence, the model can be adopted to numerically simulate the influence of various parameters on response of corroded RC beams. This would avoid the expensive laboratorial tests. Study demonstrated that experimental and analytical research are in good agreement with considered level of corrosion and corresponding deflections. Rebar yield capacity of corroded RC beam in tension zone declined, resulting in a decrease in load-carrying capability. The modelling outcomes can be used to validate the bond behaviour of Reinforced concrete cantilever beams without considering the influence of stirrups.
REVIEWING EDITOR:
1. Introduction
Reinforced concrete performance depends on design, construction methods, material selection and surrounding environment. The failure of concrete is implicated by formation of cracks which depicts the structural non-integrity that is caused under the influence environment and concrete interaction with load. Rust formation on steel surface has negative influence on concrete capacity. The passivity which is oxide layer (which cannot be destroyed easily unless acids) of rebar is due to iron oxide in the presence of high alkalinity (pH 12–13). The pH-reducing components like chlorides and carbonates gain entry into the concrete and reach the rebar surface through the pores which are interconnected. They destroy the passive layer and thus initiate deterioration of steel. The deposition of rust (hydrated ferric oxide) imposes pressure on surrounding concrete resulting in concrete spalling. This hinders the aesthetic appearance and structural integrity. Development of cracks in concrete leads to entry of aggressive agents into the concrete (Lakshmi et al., Citation2024) (the combination of steel and moisture acts as electrochemical cell). The primary reaction shown in the figure below represents corrosion initiation, which will lower the pH and thus affect the concrete quality.
It is possible to compare the electrochemical reactions that take place inside a flash battery to the electrochemical process of reinforcement corrosion (Bremner et al., 2001). The top layer of the rebar functions as an electrode made up of cathodes and anodes that are electronically connected to one another over the surface of the rebar. This rebar surface exhibits the corresponding cathodic and anodic responses. Additionally, corrosion is made possible by the complicated electrolyte interaction that occurs in the aqueous medium of the concrete’s pore water. Additionally, corrosion endangers structural safety due to a decrease in cross-sectional area and has a substantial impact on the structure’s serviceability as well as durability (Coronelli et al., Citation2012). Thickness and permeability of the concrete cover, combined with the exposure conditions, will determine how long the starting period lasts. Carbonation and chloride penetration start the corrosion.
There are two phases in the corrosion-induced degradation mechanism: initiation and deterioration. During the initial phase Aggressive media breaks the protective barrier but Functional or material weakening does not occur. Deterioration phase where Functional loss is observed (Val & Chernin, Citation2009) confirmed deterioration of concrete capacity with increase in corrosion and mathematical model was proposed to correlate loss in strength and corrosion rate (Wang et al., Citation2012) also expressed this can be very useful in continuous monitoring of the structural elements undergoing pitting and uniform corrosion. Corrosion and load play a significant role in bond strength and slip in steel concrete interphase (Xin Chai et al., Citation2021). Although chloride ions enter in one direction in actual settings, corrosion starts at the very edge of the rebar, suggesting that it does not experience homogeneous corrosion. Studies have shown, for instance, a thorough assessment of the non-uniform spread of corrosion (Du et al., Citation2006; Jang & Oh, Citation2010; Ozbolt et al., 2014).
In this FE study, the controlled beam’s material parameters were changed to simulate corrosion-induced deterioration (Kumar et al., Citation2024). Taking into account the ensuing corrosion-induced processes has the following effects: Steel area loss, decrease bond-slip characteristics, concrete cover cracking. There will be decrease in ultimate and yield capacities, depletion in the mechanical characteristics of rebar Young’s modulus and strain rate. Murcia-Delso and Shing (Citation2015) introduced a novel interface design model that simulates bond-slip behavior of rebars. The loss of bond caused by cyclic slip reversals, concrete splitting and steel yield strength are accounted for by adopting a semi-empirical law. Compression capacity of concrete will regulate the bonding capacity in case of a great quantity of transverse reinforcement. The outcomes of FE simulation on samples of lap splice and pull-out tests, revealed that splitting strength of the bond measured in a prior laboratory test, are nearly equal to the peak mean radial stress of FE measures (2-D planar FEA) (Ogura et al., Citation2008).
The FE software Abaqus had been used for modelling of steel and the concrete material (Arunkumar et al., Citation2023). The non-linearity in the RC component could be both material as well as geometric and such non-linearities are critical at a greater rate of corrosion. Empirical model by Bhargava et al. (Citation2008) co-relating bond capacity and reinforcement corrosion acknowledged the severity of bond degradation with respect to increase in corrosion. The simulation of bond was advanced by Henriques et al. (Citation2013) using the cohesive contact behaviour (the uncoupled traction-separation equation approximated the bond-slip curve). For rebars, as well as for concrete, solid 3-D element type, C3D8R was used in Abaqus CAE.
Concrete has a higher compressive strength but a lower tension strength. The linear study assumed that minor deflections and material behaviour are reflected in a linear-elastic manner. Concrete’s stress-strain behaviour under compression had been non-linear since the beginning. The investigation begins with the regulated structure, and when the load value increases, the entire theory becomes inaccurate, as the distortion causes a change in structural shape. Material nonlinearity in RC structures is difficult since both steel and concrete have nonlinear behaviour.
As per the past and present developments obtaining reliable experimental data for corrosion is challenging due to the slow and long-term nature of the corrosion process. Lack of comprehensive and reliable experimental data can hinder the validation and calibration process, making it difficult to ensure the accuracy of the corrosion models implemented in ABAQUS. This study is simulated by considering uniform corrosion based on experimental results and the results are comparable to that of FEM modelling. Similar other works are referred for this study. The effect of bar diameter and concrete cover on magnitude of rust expansion was investigated and its found that compared to experimental values numerical simulations produced higher force of rust expansion for the same cover thickness and rebar size. Similar condition is observed in this study as simulations produced greater deterioration compared to that of experimental values (Chen et al., Citation2021). Mathematical model simulating reinforcement corrosion was proposed for which non-uniform corrosion of steel reinforcement in concrete was considered to study the mechanical property deterioration. ABAQUAS and MATLAB were linked for numerical simulation of corrosion-induced cracking and it was found crack development and pattern varied for uniform and non-uniform corrosion (Zhu et al., Citation2018). Parametric studies showed drop in flexural strength with increased degree of steel corrosion (Al-Huri et al., Citation2022). Beyond 10% there will be major deterioration of bond between concrete and steel hence 10% corrosion was considered maximum (Tang et al., Citation2020).
This work proposes to investigate bond loss due to corrosion by taking the load-deflection parameter into account. Corrosion is related to bond capacity (Lakshmi et al., Citation2024). The accelerated corrosion approach is used to conduct an experimental investigation on chloride attack. To evaluate the behaviour of the beam specimen under flexure, a 3-D finite element (FE) modelling of an RC beam specimen was performed using the non-linear FE software program Abaqus CAE. It is a software capable of doing FE analysis, particularly non-linear and dynamic approaches. The plasticity damage model was used to simulate the concrete in the FE investigations. Furthermore, utilising the stress strain experimental results, all of the material behaviours required for simulation were immediately included into the selected models.
2. Control beam model to validate bond simulation approaches
The linear-elastic behaviour will not be sufficient to precisely model the RC component. In a few decades, the design of the RC structure has been transitioned from the ‘Elastic Working Stress Design’ to the ‘Ultimate Load Method’ and then to ‘Limit State Design’. The non-linearity in the RC component could be both material and geometric and such non-linearities are critical at a greater rate of corrosion. The ‘Dynamic Explicit’ type of analysis was adopted for obtaining a relatively consistent solution method that deploys explicit integration. The method had been considered on the basis that such approach is quite effective for solving the complex contact problems which are static like quasi-static mechanism model problems.
Pandit (Citation2019) used a single controlled beam sample to validate the results of multiple bond modelling methodologies. They investigated the influence of corrosion on the strength and displacement of reinforced cantilever beams (M20) made with Ordinary Portland Cement and Pozzolanic Portland Cement. Under natural conditions, the development of steel corrosion is very slow. Thus, laboratory studies need to accelerate the corrosion process, in order to achieve a short test period. This can be accomplished by applying a constant potential or an electric current of constant magnitude to the embedded steel. In accelerated tests, chloride penetration is enhanced by ion migration. Accelerated corrosion was incorporated by impressing the current between the steel in the concrete and a working electrode to study the processes of corrosion which are leading to cracking in concrete. In this practice, the samples which were made of concrete, reinforced with steel, will be immersed in a solution containing or not containing chlorides. The critical time relative to the occurrence of corrosion can be used in different ways by reducing the half-cell potential of steel or potential drop between embedded steel and a counter-electrode. These parameters represent the critical moments very similar and are related to the development of corrosion products and micro cracking. Steel corrosion was accelerated in the range from 2.5% to 10%. The rate of corrosion was measured with an ACM instrument, and a guard ring was utilised to polarise the current flow into the concrete grids. The corrosion percentage was calculated as the ratio of mass change to original mass obtained experimentally. It was discovered that there was a direct proportionality between corrosion and deflection for a given load. The study demonstrated that an ACM instrument can be used to detect corrosion rate in a non-destructive method.
The dimensions of the experimental beams are 300 mm × 400 mm × 2150 mm as shown in .
The FE model for the controlled beam was created using a basic perfect connection, in which concrete components and reinforcement were connected to surrounding interface nodes rate.
2.1. Simulation of concrete behavior
In Abaqus CAE, the concrete element was modelled as a C3D8R element, which is an eight-noded linear brick element with hourglass control and reduced integration. In contrast to an element with full integration, the element stays flexible while bending. The integration point is located in the center of the element, providing the most accurate stress and strain data at the integration points. The hourglass control eliminates zero energy modes, which prevents huge displacements from superimposing on the correct solution.
A material’s stress strain curve is processed and converted to a real stress strain curve. Because the Abaqus CAE software requires real values of stress and strain, standard values were converted, where 𝜎 and 𝜀 represent stress and strain values given by EquationEquations (1)(1)
(1) and Equation(2)
(2)
(2) .
(1)
(1)
(2)
(2)
The elastic, plastic and isotropic behaviour of concrete is highlighted in the curve. The modulus of elasticity is calculated for the model using the relation, which is 29,000 N/mm2 and 0.18 as Poisson’s ratio.
The damage plasticity model for concrete has been adopted in the analysis. The constant values for dilation angle 31° (ψ), ratio of compressive strength under biaxial loading to the uniaxial compressive strength 1.16 (fb0/fc0), invariant stress ratio 0.667 (Kc), flow potential eccentricity 0.1 (ε) and viscosity parameter 0.0 (μ) were used.
2.2. Steel behavior simulation
As with concrete, the true stress strain curve for isotropic materials should be defined in software. The stress strain curve is transformed into the real stress strain curve. The Poisson’s ratio, steel elasticity modulus, Yield stress and strain utilised in the model as mentioned in . Because the Abaqus CAE software requires true values of stress and strain, the conversion of standard values was performed using EquationEquations (1)(1)
(1) and Equation(2)
(2)
(2) from the preceding section. Reinforcing steel corrosion was achieved by assigning the degradation parameters to the rebars. Thickness loss and mechanical property degradation were assigned to the rebars based on experimental results.
Table 1. Parameters for concrete steel model.
Surface-based cohesive behaviour between the surfaces in contact is used to simulate the interaction between steel and concrete parts; one surface is the master surface and the other is the slave surface. For the concrete model, an 8-noded linear 3-D brick element (C3D8R) was used. Meanwhile, a 3-D, two-noded linear truss element (T3D2) type was used to represent the longitudinal steel reinforcing bars at the top and bottom, as well as the stirrups. The rebars and stirrups were modelled as a linear 3D two-noded truss (T3D2). Furthermore, the steel plates have been designed to prevent the problem of stress concentration at supports and loading points. The same C3D8R elements used for concrete were employed to model the supporting plates, and the perfect bond between concrete and these plates was examined. and demonstrate the discretised constituents of an RC beam for rebars and concrete.
2.3. Perfect bond case
The FE model for the controlled beam was created using a basic perfect connection, in which concrete components and reinforcement were connected to surrounding interface nodes. Following the validation of this type of bonding method with laboratory data, many different FE models of the controlled beam were developed in order to create another very dependable model using the numerous bond simulation methods. shows the load-deflection graphs of a test beam and a FE model that show significant agreement. However, it is commonly established that using the perfect bond will be enough for analysis, especially in the case of beams without corroded rebars. Nevertheless, the method was initially validated, demonstrating the confirmation of the material or other aspects of the FE model, with the only remaining task being to evaluate the bond modelling method, which first allows a realistic simulation of the controlled beam and then the beam under corrosion. The contact behaviour was defined in the normal and tangential to contact surfaces in the surface-based mechanical contact technique.
3. Corroded beam modelling
The FE model was developed before the final corroded beam model. Later the interface was built using the ‘Cohesive surface-based behaviour’ approach. Following that, the model was adjusted to account for the loss caused by corrosion. First, geometry of concrete and rebar are created. Rebars were explicitly modelled by taking truss element with corrosion-degraded properties. FE mesh was generated for concrete and rebar. Positioning of rebar was carried out according to the experimental beam. Surface-based cohesive behaviour between the surfaces in contact is used to simulate the interaction between steel and concrete parts. Material degradation criteria were used. Corroded rebar properties were defined in terms of reduced cross-sectional area, stiffness, yield strength and ultimate strength based on varying corrosion levels.
3.1. Reduced concrete cover capacity
Corrosion of rebars causes cracking in the concrete cover beneath the corroded bar, and this cracking affects beam performance, particularly in the compression zone. The model responsible for this impact was detailed in Coronelli and Gambarova (Citation2004) and it is also evident in Hanjari et al. (Citation2011) and (Biondini & Vergani, Citation2015). By-products or rust from the corrosion process will cause the volume to increase, which could cause splitting tensions and cracking of the concrete cover surrounding (Coronelli & Gambarova, Citation2004). High retention areas cause the concrete to crack, yet the intact portions within the gaps give the structure rigidity and strength. The reduced capacity of fractured concrete within the compression region caused by corrosion is given by EquationEquation (3)(3)
(3) .
(3)
(3)
where fc′ – capacity of concrete without corrosion
K – factor associated with diameter or unevenness of rebar (assumed as 0.1 in smaller diameter ribbed bars)
ε1 – mean tension strain for concrete under cracks
εo – strain for maximum compression capacity of fc′
To describe the influence of corrosion over compression, ratio of concrete capacity in the compression region to loss of steel (Xp) is based on EquationEquation (3)(3)
(3) as shown in .
The ratio of concrete capacity in the compressive region was found to decline due to corrosion with percent rise of mass loss (Xp).
Throughout this analysis, the above-mentioned modification concept was adopted for the concrete cover regions for both the upper and lower part of beam, as well as the decrease in tensile capacity (ft) of concrete were calculated on the basis of same reduction factor (Hanjari et al., Citation2011) as used for the compressive strength given by EquationEquation (4)(4)
(4) .
(4)
(4)
This study utilised bi-linear tension behaviour model, obtained from FIB Model Code (2010) for tension-crack separation relationship, after modifying crack axis with the strain results after divided it by typical length (lc). The capacity in fracture GF determined using the following EquationEquation (5)(5)
(5) .
(5)
(5)
3.2. Decrease in rebar characteristics
The corrosion leads to drop in c/s area of rebar however, this loss just is not homogeneous around the rebar. Moreover, the rate of overall decrease in cross-sectional area of rebar is equivalent to the amount of loss in mass (Xp) of the same rebar. Hence, the mean loss in the cross-sectional area could be represented by EquationEquation (6)(6)
(6) below after degradation.
(6)
(6)
where As – remaining area after corrosion.
Aso – initial rebar area prior to corrosion.
After the extensive literature survey, it was found that with the rise in corrosion, ultimate (Fuc) and the residual (Fyc) strengths of the rebar declines more than the residual area (As). Hence, along with the drop in area, there is more significant decline of yield capacity (Fy) (Du et al., Citation2006). The decline occurs owing to the non-uniform reduction or pitting corrosion.
The below EquationEquations (7)–(9) are used to modify the characteristics of steel depending on the corrosion rate
(7)
(7)
(8)
(8)
(9)
(9)
where fuc – ultimate stress dependent on initial c/s area
fyc – yield stress dependent on initial c/s area (i.e. )
ɛu – the ultimate strain
fy0 – initial value for yield capacity
fu0 – initial value for ultimate capacity
ɛ0 – ultimate elongation
Qcorr – mean sectional reduction measured as the percent of the initial quantity (equivalent to loss of mass, Xp)
𝛼y, 𝛼u & 𝛼1 – empirical factors.
The αy or αu values vary from 0 to 0.015, whereas the α1 value varies from 0 to 0.039.
4. Results and discussions
4.1. Controlled and corroded RC beam validation
For flexure and bond failure in beams, FE models were validated. For the model to validate the flexural investigation, a mesh sensitivity analysis was done. The created model is subsequently validated for bond study. represents the bending stresses of control beam and represents ultimate values for load v/s deflection obtained from experimental study. represent the comparison of deflections between FEM and experimental values for 2.5%, 5%, 7.5% and 10% corrosion, respectively.
Table 2. Ultimate values for different degrees of corrosion.
After a FE model was created to replicate a regulated or uncorroded beam, the validation process was started. The perfect bond model was initially chosen because it was simpler than other bond model techniques and accurately represented the controlled beam in terms of the results of the experiments. However, it was unable to accurately reflect the experimental investigation when employed with a corrosion-damaged model. Later, other approaches to bond interaction for the controlled and corroded models were tested. For both circumstances, the cohesive interaction strategy worked successfully. The effect of corrosion in reinforcement, cover and bond was then incorporated in the study. The graph of load versus deflection in shows a very reliable agreement between the experimental and numerical observations.
The regulated beam curve from the FE simulation increased linearly up to a load of 37 KN before significantly decreasing after that. The first crack, which releases energy when the beam has lost its elasticity and has been supported up to its maximum flexural capacity, is what caused the abrupt decrease in load. Although the bottom of the beam became weak in tension due to cracks, the loss in curve was not severe for the corroded example. The concrete cracking that first caused the beam rigidity to decline was eventually replaced by the rebar’s eventual yield. FE findings and deflection showed that the capacity of the corroded beam was lower than that of the controlled beam. As indicated in the following , additional validation was performed between two deteriorated beams with varied degrees of corrosion.
When compared to the FE results for the controlled beam, the drop in curve was significantly less in the 2.5% corroded beam. However, the curve for a 10% corroded beam by Pandit (Citation2019) shows a significant loss in bond capacity or reinforcement that has achieved its maximum yield capacity as shown in . The plot for a beam with a 10% corrosion rate shows no apparent rise or fall, which led to the conclusion above. However, many other factors, like cracks and shear, may also have an impact on the results and will be taken into consideration in future research.
The variation of the deflection value for controlled model is 4.1% from the experimental study using the cohesive surface interaction approach and about 4.9% for the corroded bond study.
4.1.1. Influence of the concrete capacity
The material parameters which are necessary for input in the model but, not available from the experimental study are then calculated from the material models recommended by CEB-FIP Model Code. The standard model code contains many important mechanical parameters for various strengths of the concrete. The bond strength for beams was obtained using the analytical model for different grades of concrete as shown in below. It could be noted that the models for greater concrete capacity and rigidity possess larger bond strength for initial stage of rebar corrosion when compared with lesser grade-assigned models. The significant increase in bond capacity with the higher grade of concrete could be resulted from more resistivity to cracks in cover of concrete and also the confining stress. represents bond strength variation with respect to concrete strength. Corrosion reduces the bond capacity of reinforced concrete. When steel corrodes, it expands, creating pressure within the concrete and causing cracking and spalling. This weakens the bond between reinforcement and concrete, decreasing the bond capacity. Higher degrees of corrosion lead to a significant reduction in bond capacity. Corrosion can also indirectly affect the compressive strength of concrete. When reinforcing steel corrodes, it undergoes loss of cross-sectional area, resulting in reduced load-carrying capacity. This loss of reinforcement can lead to reduced overall compressive strength and structural performance of the concrete. In this case (M20) grade of concrete was used and its observed that for each 2.5% increase in corrosion deflection has increased gradually lower compressive strength generally leads to increased deflection, while corrosion can increase deflection and weaken the compressive strength.
4.2. Analyses for mesh sensitivity
To study the influence of mesh sizes, the same FE models validated earlier are adopted with varied sizes of mesh for different parts of beam assembly. In the first approach, the mesh size for both concrete and steel parts were modified for three distinct sizes of 8, 25 and 40 mm. shows that the outcomes of Abaqus analyses for three different mesh sizes did not considerably alter the results.
In Abaqus modelling, sensitivity analysis refers to the study of how changes in certain input parameters, such as mesh size or mesh type, affects the results of a simulation. Sensitivity analysis helps engineers and analysts understand the impact of these parameters on the model’s behavior and make informed decisions about the simulation setup. Mesh size refers to the element size or the characteristic size of the elements used to discretise the geometry in a FE model. Conducting sensitivity analysis for mesh size involves varying the element size and observing the corresponding changes in the simulation results.
Based on the sensitivity analysis, appropriate mesh size was selected that balanced computational efficiency and accuracy. Considering a mesh size that provided converged results and minimised significant changes in the output quantities with further refinement was adopted.
The study has been carried out in two distinct approaches:
The mesh size was maintained equal for both concrete and the reinforcement parts.
The mesh size of concrete and reinforcement were kept unequal.
Furthermore, it is understood that it would be better for the study if the mesh sizes for the steel and concrete were identical or slightly closer. When compared to mesh sizes of 40 mm, the 25 mm mesh size did somewhat improve the outcomes. Of 25 mm mesh size was used for the research. As crack path and position are mesh dependent and this study is in agreement with the previous study of (B Sanz et al., Citation2013).
In the other approach, the same FE model of beam with 2.5% corrosion was used for validation. and show deformation models for considered mesh variations. The FE model was incorporated with varied mesh sizes for concrete and steel.
The results obtained using this technique is as shown in , It is observed that on providing of varying mesh sizes for concrete and steel led to increase in deflection for constant load range (20 kN–30 kN) and the curve pattern for varying mesh sizes was not in agreement with the experimental results of Pandit (Citation2019).
4.3. Parametric study
The parametric analyses have been performed in order to understand the influence of different parameters on bond behaviour and the residual capacity of the beams when subjected to distinct levels of corrosion. The analytical and FE models constructed and validated in the above studies were used for this study. The parameters required for analytical study of capacity or deterioration of bond in control beam models which were experimentally tested by Pandit (Citation2019) are as tabulated in below. represents the model of exponential decay in friction (Abaqus CAE Manual A, Citation2013).
Figure 19. Model of exponential decay in friction (Abaqus CAE Manual A, Citation2013).

Table 3. Parameters used for analytical model.
The transition among these two correlative coefficient values could be worked out in Abaqus by adopting an exponentially decaying relation under which the value of coefficient of friction drops exponentially in accordance with the equation below:
(10)
(10)
where
– coefficient for kinetic friction
– coefficient for static friction
dc – decay factor (user-defined)
– rate of slip.
4.3.1. Influence of corrosion damages on FE model
In this FE analysis, two different damages induced by corrosion were incorporated as listed below:
Reduction in c/s area of steel rebar.
Deterioration of Bond.
The boundary conditions between concrete and steel typically determine crack patterns. One of the two corrosion damage parameters mentioned above was only included in the model at a time during the FE study. One at a time consideration made it simpler to determine the unique effects of damage on the flexural behaviour of FE models. For this parametric research, the 2.5% corroded FE beam model proven above was used. After that, FE analyses were carried out as shown below:
First, only 2.5% reduction in c/s area of steel rebar part (marked as FE2.5% area).
Second, the bond deterioration parameters for 2.5% corroded beam were only specified in the model (marked as FE2.5% bond).
Later, multiple corrosion damage factors were simultaneously incorporated to FE model (marked as FE2.5% all).
and represent the steel cage deformation and crack propagation for 2.5% corrosion. The laboratory results for the control beam and the beam with 2.5% corrosion are shown in . FE analyses for controlled beam, c/s area decrease, bond deterioration and ultimately, the FE analysis for all corrosion-related damages when specified simultaneously in the model.
Stress analysis involved the determination of internal stresses within a material or structure under applied loads. These stresses were important as they can influence the behaviour and integrity of the material. In order to correlate the experimental and FEM models, comparison of stress and strain values were made from the data obtained from both methods. The experimental data acted as reference values for stress and strain at different levels of cracking. These values were compared with the corresponding stress and strain values obtained from the FEM model. The goal was to minimise the differences between the two sets of results and achieve a reliable prediction of the behaviour of the cracked structure under various loading conditions. Overall, stress analysis and crack analysis, along with the correlation of experimental and FEM models, provided valuable insights into the behaviour of cracked materials or structures. The flexural response of the 2.5% corroded beam in the FE analysis did not deviate significantly from the experimental research on the control specimen till yield limit. However, the reduction in rebar cross-section would only have a substantial impact on the flexural behaviour once the beam reached its yield limit. Therefore, until the yield limit, there was little difference between the control and corroded beams.
The results of FE2.5 percent Bond demonstrated a significant. FE 2.5% area and FE 2.5% represent the deterioration property applied to the rebar in terms of area loss and bond strength loss respectively to represent corrosion. Load versus deflection for this criterion is represented in .
Degradation in bond strength. Further, it could be inferred from the study that the structural deterioration of RC Beams induced by corrosion is mainly due to the reduction in bond capacity. The results of FE analysis (for FE2.5%All) indicate the reduction in both stiffness and the capacity. The volume expansion of rebar deteriorated the bond between concrete and the rebar which is in accordance with the study of El Alami et al. (Citation2021).
5. Conclusions
The 3-D FE modelling techniques were incorporated to construct both un-corroded (controlled) and corroded RC beam models. For non-linear FE model in Abaqus, the standard technique of analysis and plastic damage model for concrete was used to reflect the corrosion. Ultimately, the cohesive behavior method yielded considerably acceptable outcomes in both controlled and the corroded beams. The effect of corrosion in reinforcement, cover and bond was then incorporated in the study. The mesh sensitivity study was carried out with same and varied mesh sizes for both concrete and steel parts to ascertain the influence over FE results and also to adopt the optimum mesh size for this study. Further, the parametric study was performed on both analytical and FE models to ascertain the influence of different grades of concrete, reduction in c/s area of rebar and deterioration of the bond on flexural capacity and bond behaviour of RC Beam.
The study is limited for uniform corrosion losses only and pitting corrosion cannot be simulated, future trials can be carried out to simulate pitting corrosion. Abaqus does not have built-in corrosion models, so there is a need to include user-defined functions to simulate pitting corrosion. Using other software corrosion modelling can be done and later integrated with Abaqus.
Following are the observations made from the analysis:
The reaction of longer beams with an acceptable number of rebars would not be changed by a modest reduction in the bond capacity.
The load-deflection curve showed considerable agreement between FE analyses and experimental findings.
The developed FE model of RC beam under corrosion could be adopted to obtain the load at failure with considerable accuracy. Hence, the model be adopted to numerically simulate the influence of various parameters on response of corroded RC beams. This would avoid the expensive laboratorial tests.
Maintaining uniform mesh size for steel and concrete is necessary as varying mesh size influences the load-deflection relation.
The absolute bond loss between the concrete and rebars is likely the most serious damage caused by corrosion.
Rebar yield capacity of corroded RC beam in tension zone declines, resulting in a decrease in load-carrying capability.
Future scope
The work could be extended with 3-D solid element for both stirrups and rebars and also, the effect of interfacial cracks due to volumetric expansion of rust on beam performance.
FE model preparation can also be done for beam subjected to pitting corrosion.
Author details
Aishwarya Lakshmi1, Poornachandra Pandit2*, Arun Kumar Y M2, Yamuna Bhagwat1, Gopinath Nayak3, Amogh Shetty4
1Research Scholar, 2Assistant Professor, 3Associate Professor, 4Post Graduate Student
1,2,3,4Department of Civil Engineering, Manipal Institute of Technology, Manipal Academy of Higher Education, Manipal, India;
*Corresponding author: [email protected]
Disclosure statement
No potential conflict of interest was reported by the author(s).
Additional information
Notes on contributors
Aishwarya Lakshmi
Aishwarya Lakshmi received her Master of Technology in Construction Technology from NMAM Institute of Technology, Nitte, India. She is currently pursuing her Ph.D in Department of Civil Engineering, Manipal Institute of Technology, Manipal Academy of Higher Education, Manipal, India. Her research interests include concrete technology, durability of concrete structures.
Poornachandra Pandit
Poornachandra Pandit received his Master of Technology in Structural Engineering and Ph.D from National Institute of Technology, Surathkal, Karnataka, India. Presently he is working as an Assistant Professor Senior scale in the department of Civil Engineering, Manipal Institute of Technology, Manipal Academy of Higher Education, Manipal, India. His research interests include concrete technology, Reinforcement corrosion, Finite Element Modeling, Durability of concrete structure, Structural engineering, Corrosion of Alkali Activated Concrete, Durability of Alkali Activated Concrete and Effect of temperature on Alkali Activated Concrete. Currently he has been guiding 6 PhD Students. He has published several papers in reputed journals and conferences.
Arun Kumar Y.M.
Arun Kumar Y. M. received his Master of Technology in Structural Engineering and pursuing Ph.D in Department of Civil Engineering, Manipal Institute of Technology, Manipal Academy of Higher Education, Manipal, India Presently he is working as an Assistant Professor Senior scale in the department of Civil Engineering, Manipal Institute of Technology, Manipal Academy of Higher Education, Manipal, India. His research interests include Soil structure interaction, Finite Element modeling, Structural engineering, Pile foundation.
Yamuna Bhagwat
Yamuna Bhagwat received her Master of Technology in Structural Engineering from KLE DR. M. S. Sheshgiri College of Engineering and Technology, Belagavi, India. She is currently pursuing her Ph.D in Department of Civil Engineering, Manipal Institute of Technology, Manipal Academy of Higher Education, Manipal, India. Her research interests include concrete technology, durability of concrete structures, structural analysis, artificial intelligence and optimization algorithms.
Gopinath Nayak
Gopinatha Nayak received his Master of Technology in Structural Engineering and Ph.D from National Institute of Technology, Surathkal, Karnataka, India. Presently he is working as a Professor in the department of Civil Engineering, Manipal Institute of Technology, Manipal Academy of Higher Education, Manipal, India. He has published several papers in reputed journals and conferences. Currently he has been guiding 9 Ph.D students. His research interests include concrete technology, recycling plastic wastes, Durability of concrete structure, Structural engineering.
Amogh Shetty
Amogh Shetty received his Master degree in Structural Engineering from Department of Civil Engineering, Manipal Institute of Technology, Manipal Academy of Higher Education, Manipal, India. His research area includes Corrosion of RC beams, Finite Element modeling.
References
- Abaqus CAE Manual, A. (2013). Abaqus CAE analysis user’s manual version 6.13. Dassault Systèmes Simulia Corp.
- Al-Huri, M. A., Al-Osta, M. A., & Ahmad, S. (2022). Finite element modelling of corrosion-damaged RC beams strengthened using the UHPC layers. Materials, 15(21), 7606. https://doi.org/10.3390/ma15217606
- Arunkumar, Y. M.,Prashanth, S.,Pandit, P.,Girish, M. G., &Shetty, A. (2023). Finite element analysis of bond behavior in corroded reinforced concrete beams: State-of-the-art. Journal of Applied Engineering Science, 21(4), 1031–1042. https://doi.org/10.5937/jaes0-42252
- Bhargava, K., Ghosh, A. K., Mori, Y., & Ramanujam, S. (2008). Suggested empirical models for corrosion-induced bond degradation in reinforced concrete. Journal of Structural Engineering, 134(2), 221–230.) https://doi.org/10.1061/(ASCE)0733-9445(2008)134:2(221)
- Biondini, F., & Vergani, M. (2015). Deteriorating beam finite element for nonlinear analysis of concrete structures under corrosion. Structure and Infrastructure Engineering, 11(4), 519–532. https://doi.org/10.1080/15732479.2014.951863
- Chai, X., Shang, H., & Zhang, C. (2021). Bond behavior between corroded steel bar and concrete under sustained load. Construction and Building Materials, 310, 125122. https://doi.org/10.1016/j.conbuildmat.2021.125122
- Chen, F. X., Zhong, Y. C., Gao, X. Y., Jin, Z. Q., Wang, E. D., Zhu, F. P., Shao, X. X., & He, X.Y. (2021). Non-uniform model of relationship between surface strain and rust expansion force of reinforced concrete. Scientific Reports, 11(1), 8741. https://doi.org/10.1038/s41598-021-88146-2
- Coronelli, D., & Gambarova, P. (2004). Structural assessment of corroded reinforced concrete beams: modelling guidelines. Journal of Structural Engineering, 130(8), 1214–1224.) https://doi.org/10.1061/(ASCE)0733-9445(2004)130:8(1214)
- Coronelli, D., Hanjari, K. Z., & Lundgren, K. (2012). Severely corroded RC with cover cracking. Journal of Structural Engineering, 139(2), 221–232. https://doi.org/10.1061/(ASCE)ST.1943-541X.0000633
- Du, Y. G., Chan, A. H. C., & Clark, L. A. (2006). Finite element analysis of the effects of radial expansion of corroded reinforcement. Computers & Structures, 84(13–14), 917–929. https://doi.org/10.1016/j.compstruc.2006.02.012
- El Alami, E., Fekak, F. E., Garibaldi, L., & Elkhalfi, A. (2021). A numerical study of pitting corrosion in reinforced concrete structures. Journal of Building Engineering, 43, 102789. https://doi.org/10.1016/j.jobe.2021.102789
- Hanjari, K. Z., Kettil, P., & Lundgren, K. (2011). Analysis of mechanical behavior of corroded reinforced concrete structures. ACI Structural Journal, 108(5), 532–541.
- Henriques, J., Simões, Silva, L., & Valente, I. B. (2013). Numerical modeling of composite beam to reinforced concrete wall joints. Part I: Calibration of joint components. Engineering Structures, 52, 747–761. https://doi.org/10.1016/j.engstruct.2013.03.041
- Jang, B. S., & Oh, B. H. (2010). Effects of non-uniform corrosion on the cracking and service life of reinforced concrete structures. Cement and Concrete Research, 40(9), 1441–1450. https://doi.org/10.1016/j.cemconres.2010.03.018
- Kumar, A.,Shetty, K. K., &Krishnamoorthy, A. (2024). Efficiency of RCC piles with helical grooves subjected to axial and lateral loads in cohesionless soil. Cogent Engineering, 11(1), 15.
- Lakshmi, A.,Pandit, P.,Nayak, G.,Bhagwat, Y., &Gundlapalli, P. (2024). Performance evaluation of low volume synthetic fibres in pozzolanic cement concrete. Cogent Engineering, 11(1). https://doi.org/10.1080/23311916.2024.2319398
- Lakshmi, A.,Pandit, P.,Nayak, G.,Bhagwat, Y., &Kumar, S. (2024). Influence of corrosion-based section loss on morphology and tensile capacity of pre-stressing strands. Journal of Structural Integrity and Maintenance, 9(1). https://doi.org/10.1080/24705314.2024.2302655
- Murcia-Delso, J., & Shing, P. (2015). Bond-slip model for detailed finite-element analysis of reinforced concrete structures. Journal of Structural Engineering, 141(4), 1–10. https://doi.org/10.1061/(ASCE)ST.1943-541X.0001070
- Ogura, N., Bolander, J. E., & Ichinose, T. (2008). Analysis of bond splitting failure of deformed bars within structural concrete. Engineering Structures, 30(2), 428–435. https://doi.org/10.1016/j.engstruct.2007.04.004
- Ožbolt, J., Oršanić, F., & Balabanić, G. (2014). Modeling pull-out resistance of corroded reinforcement in concrete: Coupled three-dimensional finite element model. Cement and Concrete Composites, 46, 41–55. https://doi.org/10.1016/j.cemconcomp.2013.10.014
- Pandit, P. (2019). Experimental study on accelerated corrosion technique of OPC and PPC beams in coastal environment. Journal of Corrosion Science and Engineering, 22.
- Sanz, B., Planas, J., & Sancho, J. M. (2013). An experimental and numerical study of the pattern of cracking of concrete due to steel reinforcement corrosion. Engineering Fracture Mechanics, 114, 26–41. https://doi.org/10.1016/j.engfracmech.2013.10.013
- Tang, H., Peng, J., Xiao, L., Liu, X., & Zhang, J. (2020). Numerical simulation of corroded reinforced concrete beam strengthened by a steel plate with different strengthening schemes. Advances in Civil Engineering, 2020, 1–19. https://doi.org/10.1155/2020/4236943
- Val, D. V., & Chernin, L. (2009). Serviceability reliability of reinforced concrete beams with corroded reinforcement. Journal of Structural Engineering, 135(8), 896–905.) https://doi.org/10.1061/(ASCE)0733-9445(2009)135:8(896)
- Wang, L., Ma, Y., Zhang, J., & Liu, Y. (2012). Probabilistic analysis of corrosion of reinforcement in RC bridges considering fuzziness and randomness. Journal of Structural Engineering, 139(9), 1529–1540. https://doi.org/10.1061/(ASCE)ST.1943-541X.0000738
- Zhu, X., Meng, Z., Liu, Y., Xu, L., & Chen, Z. (2018). Entire process simulation of corrosion due to the ingress of chloride ions and CO2 in concrete. Advances in Materials Science and Engineering, 2018, 1–12. https://doi.org/10.1155/2018/9254865