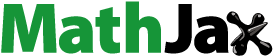
ABSTRACT
Asymmetric colloidal Janus particles (JPs) have stimulated research which spans many disciplines, often due to possibilities for their assembly into larger structures. This review aims to identify opportunities for physicists and others working on spherical JPs clustering in bulk fluid, which is a simple and fundamental type of JP assembly. The review focuses on JPs with two hemispheres with differing surface properties but also considers ‘patchy’ particles with smaller or more numerous distinct surface areas. Research to date has featured many simulation methods, including point potentials with angular dependence, multi-site approaches, and detailed numerical techniques. The field lacks experiments and 3D simulations which allow clear quantitative comparisons and hence validation of fundamental principles, including the behaviour of so-called ‘floppy’ bonds between two or more JPs. Research into non-equilibrium clustering and the role of hydrodynamics in assembly will also be of ongoing interest, because these topics will be significant in any practical assembly strategy.
1. Introduction
Janus particles (JPs) are colloids that have two distinct physical or chemical surface properties – a tangible representation of the Roman deity Janus, a god of doors, gates, and transitions. Fabrication protocols for Janus-like polymer discs were explored as early as 1961 [Citation1], and the term ‘Janus beads’ was first used more than three decades ago to discuss particles with polar and apolar sides forming interesting films at an air-water interface [Citation2,Citation3]. The study of JPs has strongly accelerated more recently, fuelled by advances in methods for advanced materials science [Citation4–6]. There is a near-limitless variety of JPs [Citation7–9]. The archetypal JP is a sphere divided into hemispheres with different surface chemistries [Citation10,Citation11], and chemical patterning can also include (for example) JPs with smaller chemically modified areas known as ‘patchy’ particles [Citation12,Citation13], or even stripes [Citation14]. Examples of non-spherical JPs include snowmen [Citation15,Citation16], discs [Citation17,Citation18], rods [Citation19,Citation20], dumbbells [Citation21], and half-raspberries [Citation22,Citation23]. A large and growing range of JP synthesis strategies has regularly been the focus of reviews [Citation24–32].
The anisotropy of JPs suggests many interesting applications [Citation9,Citation27–33]. For example, many authors have worked on JPs for biomedical applications, especially imaging and sensing, which are aided by the versatile multifunctional nature of JPs [Citation34–38]. Drug delivery systems could also use JPs by coupling self-propulsion, recognition, or triggering functions with the drug cargo [Citation38–41]. Marschelke et al. [Citation42] have reviewed potential environmental benefits of using JPs, from sensing and monitoring functions through to water decontamination. Various JP types have also been studied for applications involving catalysis [Citation43–46], optics [Citation47–53], interfacial rheology [Citation54,Citation55], and stabilisation of emulsions [Citation56,Citation57].
Assembly of interesting materials consisting of many-particle clusters and phases is an important aspect of many promising applications [Citation33]. JP assembly can be described as a form of supramolecular chemistry with bonds formed between the particles [Citation24,Citation58,Citation59], so that JPs can be building blocks in structures that would not be possible for isotropic particles [Citation7,Citation60]. JP self-assembly – referring to the spontaneous formation of organised structures through a reversible, stochastic process [Citation61] – can be manipulated via a particle’s design and environmental factors, leading to controlled assembly and ‘designing’ for outcomes [Citation51–53,Citation59,Citation62–65]. These ideas are somewhat inspired by natural systems such as proteins and DNA which use self-assembly to construct nanoscale architectures [Citation66–69].
This review is most concerned with fundamental aspects of Janus sphere self-assembly in 3D bulk solution. Small clusters of JPs (2, 3, and more) are of most interest because these systems and their dynamics underpin any larger assemblies, so that they provide ongoing opportunities for interdisciplinary research. Topics such as the orientation-dependent interactions between JPs and the dynamics of cluster assembly are practically relevant for applications involving JP self-assembly, but lack experimentally validated descriptions. JPs with distinct hemispheres are emphasised because they can be made using the simplest and most common experimental route, via directional deposition of a metallic layer onto micrometre-scale spheres with subsequent chemical modification for solvophobicity. However, the review is also concerned with spherical ‘patchy’ particles with any number and geometry of distinct surface areas – e.g. Duguet et al. have studied particles with up to 10 patches [Citation4]. Patchy particles include inverse-patchy colloids (IPCs) [Citation26] which (unlike solvophobic JPs) have mutual attraction between different areas, e.g. they feature electronic charge, or complementary DNA [Citation70] and protein [Citation71] interactions. The most relevant previous reviews have addressed the general topic of JP self-assembly [Citation4,Citation26,Citation28,Citation31,Citation59,Citation63,Citation72], while predictive modelling and simulation of colloidal assembly have recently been addressed without focusing on spherical JPs [Citation33,Citation73].
The review does consider hydrodynamics, because effects such as shear flows and diffusion are likely to be significant in any practical assembly process. Studies of 2D assemblies [Citation63] (i.e. influenced by surfaces or interfaces) are not the main topic for this review, but they are mentioned where they have contributed to our general understanding of JP interactions. Other popular related topics are not emphasised in order to avoid introducing extra complexity and experimental constraints, although examples are discussed where they are instructive. Such topics include non-spherical JPs [Citation33], assembly that is ‘directed’ by applied fields (e.g. electromagnetic [Citation31,Citation33], or optical), and active (self-propelling) JPs [Citation74,Citation75]. Janus nanoparticles are in scope but are more likely to be non-spherical as the continuum limit approaches. They are also more influenced by Brownian motion and harder to observe than micrometre-scale JPs, and the latter have produced most of the relevant experimental observations to date.
The review first recounts the reported range of theoretical and simulation approaches to studying assembly. The next section addresses research into hydrodynamics, kinetically stabilised structures, and the phenomenon known as flexible (or ‘floppy’) bonding. We then review the various ways that assembly of Janus spheres has been studied experimentally, focussing on those studies which feature comparisons with theory and simulations, and the final section includes a summary of areas in which research has not been comprehensive to date, providing opportunities for ongoing work. A key theme is the opportunity for interdisciplinary research to link theory with experiments, develop fundamental understanding, and thus contribute to the design and development of JPs and handling strategies contributing to applications.
2. Janus particle interactions: simulations and theory
Interactions between two or more JPs have been studied using a considerable range of simulation and theoretical techniques. The methods used can be put into three main categories: firstly simulations that consider JPs to be point potentials (equivalent to single-site potentials), secondly simulations that model JPs as rigid spheres with multiple interaction sites distributed over their surfaces, and thirdly other numerical and theoretical approaches. Simulations have typically used conventional many-body techniques such as Monte Carlo (MC), molecular dynamics (MD) and Brownian dynamics (BD). Generally, computational cost increases with the level of detail of the simulated interaction between JPs. Simulations have therefore ranged from detailed studies of one or two JPs, to analysis of several-JP clusters, to finding and classifying phase diagrams [Citation76] for many JPs – typically as a function of concentration, and of interaction strength relative to thermal energy. Various technical methods have been used to identify structures, including correlation functions, order parameters, and radial distribution functions [Citation77–80]. As this review is focussed on interactions within small clusters, we do not include detailed catalogues of large-scale structures. For brevity, we omit some of the more technical aspects of simulations (e.g. the thermostat or boundary conditions used).
2.1. Point potentials
Potentials representing JPs as points are typically based on methods established for isotropic colloids, with directional dependence added [Citation33,Citation85]. Four prominent examples of point interactions between pairs of single-patch JPs are plotted in . These interactions are mathematically described in Supplementary Material (Table S1) using the geometric definitions shown in [Citation81]. The centres of particles i and j are separated by a vector with magnitude r, and the vectors
and angles
are defined relative to a pole passing through the centre of each particle’s hydrophobic (unless stated) patch. Some patches are defined by an area covered by the polar angle β. Each particle is a sphere of diameter σ, with ϵ indicating the depth of a potential well. The interaction range is treated differently in the various models, but its length scale is generally λσ for some constant λ, with λD specifically denoting the Debye length, while θtail, C1, C2, ρ1 and ρ2 are constants for specific cases discussed below. Symbols and signs may differ from the original papers.
Figure 1. Point potentials used in JP assembly studies, detailed in supplementary material, table S1. (a) Typical geometric definitions for point potentials. Reproduced with permission from [Citation81], conveyed through Copyright Clearance Center, Inc. (b) Radial variation of contributions to potentials. (c) Angular variation of contributions to potentials. Calculations use specific values from corresponding KF [Citation82] (potential form , λ = 1.5,
), LJ [Citation83] (
,
,
), Yukawa [Citation77] (
,
, λ = 3,
) and DLVO [Citation84] (
,
,
, λ = 1.05, ρ1 = 1,
) studies. Values for C1 and C2 are defined here arbitrarily, and for Hong et al.’s model [Citation84] note that
when
and hydrophobic sides are facing.
![Figure 1. Point potentials used in JP assembly studies, detailed in supplementary material, table S1. (a) Typical geometric definitions for point potentials. Reproduced with permission from [Citation81], conveyed through Copyright Clearance Center, Inc. (b) Radial variation of contributions to potentials. (c) Angular variation of contributions to potentials. Calculations use specific values from corresponding KF [Citation82] (potential form Vf(θi)f(θj), λ = 1.5, β=90∘), LJ [Citation83] (VR+VAfA(θi)fA(θj), θtail=10∘, β=90∘), Yukawa [Citation77] (VR+VAfA(θi,θj), C1=ϵ, λ = 3, θj=0) and DLVO [Citation84] (VRfR(θi)fR(θj)+VAfA(θi)fA(θj), C2=ϵ, λD=σ/100, λ = 1.05, ρ1 = 1, ρ2=−1) studies. Values for C1 and C2 are defined here arbitrarily, and for Hong et al.’s model [Citation84] note that fR(θ)=0 when r>λσ and hydrophobic sides are facing.](/cms/asset/f4401f4a-ae4d-4687-855e-d347eefcb9ee/tapx_a_2341759_f0001_oc.jpg)
Simulations of 3D JP assemblies have been most commonly [Citation53,Citation78,Citation86–96] performed with MC methods using the Kern-Frenkel (KF) potential [Citation82], which models JPs as impenetrable hard spheres. Two spheres attract each other via a square well potential when their patches are facing and . Parameters varied in simulations have included the attractive patch size [Citation86,Citation87,Citation90] and the interaction length λ (e.g. from λ = 1.05 [Citation86] to 1.20 [Citation88], to 1.50 [Citation87], and systematic variation across the range
[Citation95]). The same method has been used to study JPs with more than one patch, such as triblock [Citation78,Citation92] and rectangular-patch [Citation53] JPs. The topics studied have included the boundary between gas-like (micellar) and liquid-like phases [Citation89], variations in 1D and 2D micelle structures [Citation87], formation of open crystal structures at low (30%) patch coverage [Citation86], and close packing giving way to sheets, lamellae and lattices at λ > 1.20 [Citation95]. Based on KF simulations, phase diagrams have been drawn using free energy calculations [Citation88], cluster sizes and structures have been studied [Citation90], and comparisons have been made with statistical mechanics calculations [Citation91].
Discontinuous features of the KF potential (the hard sphere, square well, and boundary between surface areas) have been addressed in several studies [Citation83,Citation97–99] using the form of the Lennard-Jones (LJ) potential, which is appropriate for relatively weak, short-ranged interactions such as van der Waals forces. Miller et al. [Citation83] simulated aggregates () using a potential that included an isotropic ‘soft’ Weeks – Chandler – Anderson (WCA) repulsion [Citation33], i.e. a truncated and shifted LJ potential. The attractive term was also a LJ potential, but with the interaction decaying to zero over at the patch edges.
Figure 2. (a) Examples of JP clusters simulated using a LJ point potential. From left to right with increasing β: small micelles (4–13 particles), branched worm-like aggregates, and fluid amorphous aggregates. Reproduced with permission from [Citation83], copyright (2009) by the American Physical Society. (b) Examples of n-particle clusters simulated using a Yukawa-like potential. Reproduced with permission from [Citation77], AIP Publishing. (c) Staged assembly strategies using triblock patchy particles, simulated using a hydrophobic/charged point potential. Reproduced with permission from [Citation50], copyright (2018) by the American Chemical Society.
![Figure 2. (a) Examples of JP clusters simulated using a LJ point potential. From left to right with increasing β: small micelles (4–13 particles), branched worm-like aggregates, and fluid amorphous aggregates. Reproduced with permission from [Citation83], copyright (2009) by the American Physical Society. (b) Examples of n-particle clusters simulated using a Yukawa-like potential. Reproduced with permission from [Citation77], AIP Publishing. (c) Staged assembly strategies using triblock patchy particles, simulated using a hydrophobic/charged point potential. Reproduced with permission from [Citation50], copyright (2018) by the American Chemical Society.](/cms/asset/f63381be-5aab-4caf-a155-f6ece6e6b17a/tapx_a_2341759_f0002_oc.jpg)
Rosenthal et al. [Citation77] created a pair potential consisting of an isotropic soft (WCA-like) repulsion term added to an attractive Yukawa potential with orientation dependence, arranged so that the attractive force is maximised when hydrophobic sides face each other. MD simulations identified a region in temperature-density space in which clusters evolved from near-spherical aggregates () through to linked 13-particle icosahedra. Similar potentials have often been used to study JPs subject to shear and confinement [Citation85,Citation100–102]. The Yukawa potential is also naturally suited for modelling IPCs with areas of opposing electronic charge [Citation71,Citation103] because it was developed for screened electrostatic interactions [Citation33].
Hong et al.’s MC simulations for hemispherical JPs [Citation84] used a point potential with two components, each having angular dependence. Hydrophobic attraction between patches was mimicked using a short-ranged (λ = 1.05) square well tapering smoothly with orientation towards zero at the patch edge. A repulsive electrostatic interaction based on the Derjaguin-Landau-Verwey-Overbeek (DLVO) potential had surface charge densities off (ρ1) and on (ρ2) the hydrophobic patch, and a magnitude (C2) that can be linked to physical quantities. If aggregation was not prevented by electrostatic repulsion, clusters formed and merged into worm-like chains with increasing particle concentration. Other studies that have simulated JPs with one hydrophobic and one charged hemisphere have included JPs that aggregate into helices within a cylindrical ‘trap’ [Citation104], and JPs represented by a potential with an attractive term decreasing smoothly from a maximum at pole-to-pole orientation () to zero when patches are not in contact [Citation81,Citation105]. A similar angular dependence has been employed in MC and BD simulation studies of spherical triblock particles [Citation50,Citation52]. These studies have demonstrated methods for hierarchical assembly () by first forming tetrahedral and octahedral clusters, then using a change in temperature to form cubic diamond and body-centered cubic crystals [Citation50] or tetrastacks [Citation52], with possible applications in photonics.
2.2. Multi-site rigid models
Geometries can be investigated in greater detail by representing JPs as rigid assemblies of smaller ‘sites’ (or ‘points’, or ‘atoms’) distributed over the particle’s surface. Examples with as few as three sites have been reported for 2D [Citation111] and 3D [Citation112] systems. The latter study used a sum of overlapping apparent sphere volumes to determine the weighting of each interaction, achieving reasonable correspondence with a fully analytic method for IPCs. BD has been a popular method for multi-site modelling of bipolar Janus spheres and one-patch IPCs [Citation113–116]. Ninety-two-bead JPs interacting via a DLVO potential produced a greater number of elongated aggregates for small patches (relative to λD) [Citation113], assemblies of up to ~ 120 patchy particles were studied for JPs of radius 2.5σ [Citation114], while self-assembly of Janus ellipsoids [Citation115] and triblock patchy particles [Citation116] have also been studied.
Multi-site rigid JPs have also been studied using MC [Citation110] and MD. An MD study with 60-site Coulombic JPs, in solvent modelled as neutral hard spheres, produced rings and strings (), with the latter tending to form at higher electrolyte concentrations and for larger particles. Another MD study [Citation79] demonstrated that multiple sites can be used for coarse-grained models of molecules such as alkane chains. More recently, multi-site structures have encouraged explicit solvent interactions to be included in MD simulations. Safaei et al. [Citation107,Citation117,Citation118] studied the hydrodynamics of amphiphilic LJ Janus spheres with radii up to 10σ (). Kharazmi et al. studied diffusion of a JP in an LJ fluid, with the particle modelled as a convex polyhedron () within a sphere of radius 2.08σ [Citation108]. Multiparticle collision dynamics (MPCD) allowed the inclusion of explicit solvent in a study of 162-site JPs (), with hydrophobic and hydrophilic sites having LJ and purely repulsive WCA interactions, respectively [Citation109]. MD studies have also been carried out using LJ potentials acting between sites on JPs and nearby walls [Citation119,Citation120].
Figure 3. (a) Rings and strings formed by 60-site coulombic JPs. Reproduced with permission from [Citation106], conveyed through Copyright Clearance Center, Inc. (b) Multi-site LJ JPs in MD simulations of a dimer, showing (left to right) the geometric setup, the probability distribution for polar angles with examples of specific configurations labelled (i)–(iii), and comparison between MD and numerical integration (NI) occurrence probability results. Reproduced with permission from [Citation107], copyright (2021) by the American Physical Society. (c) A 72-vertex multi-site JP structure, reproduced with permission from [Citation108], with the permission of AIP Publishing. (d) Multi-site JP with radius 3σ and . Reproduced with permission from [Citation109], copyright (2020) American Chemical Society. (e) Geometry (left) and potential landscape (right) for calculations of Coulombic interactions between JPs with 12,002 sites. Reproduced with permission from [Citation110], copyright (2006) American Chemical Society.
![Figure 3. (a) Rings and strings formed by 60-site coulombic JPs. Reproduced with permission from [Citation106], conveyed through Copyright Clearance Center, Inc. (b) Multi-site LJ JPs in MD simulations of a dimer, showing (left to right) the geometric setup, the probability distribution for polar angles with examples of specific configurations labelled (i)–(iii), and comparison between MD and numerical integration (NI) occurrence probability results. Reproduced with permission from [Citation107], copyright (2021) by the American Physical Society. (c) A 72-vertex multi-site JP structure, reproduced with permission from [Citation108], with the permission of AIP Publishing. (d) Multi-site JP with radius 3σ and β=90∘. Reproduced with permission from [Citation109], copyright (2020) American Chemical Society. (e) Geometry (left) and potential landscape (right) for calculations of Coulombic interactions between JPs with 12,002 sites. Reproduced with permission from [Citation110], copyright (2006) American Chemical Society.](/cms/asset/f71665c1-3ba4-4a6e-a979-78035f1e1683/tapx_a_2341759_f0003_oc.jpg)
2.3. Numerical integration and analytic results
In the limit of very many ‘sites’, multi-site approaches become numerical integration methods. JP models featuring > 1000 sites have typically used electrostatic potentials, with solvent implicitly accounted for via the screening length [Citation110,Citation121–123]. Hong et al. modelled Janus spheres constructed from 12,002 charged points [Citation110] each with a square well potential representing a screened electrostatic interaction. This approach was used to calculate the interaction potential as a function of sphere orientation ()). Another study of hemispherical JPs with > 1000 point charges used a Coulombic pair potential which represents a defined charge and has a smooth form suitable for MD [Citation122]. Optimised algorithms were used in large MC and MD simulations [Citation122,Citation123], providing data on equilibrium cluster configurations. It was recently reported that this numerical method produces good agreement with a more computationally efficient analytic approach based on the Derjaguin approximation [Citation124]. 1684-site amphiphilic spheres were used in a dissipative particle dynamics study of JPs confined in nanotubes with explicit solvent [Citation125]. Geometric landscapes for JP DLVO interactions have also been calculated using a detailed tensorial approach [Citation126], and a simplified pair potential generated from the results was used in MC simulations to find low energy configurations for clusters ranging from 3 to 14 JPs.
A ‘continuous’ numerical integration method has been developed for JP interactions using the LJ potential and including explicit solvent [Citation107]. The approach is based on an analytical equivalent that has been applied to non-Janus LJ nanostructures [Citation127,Citation128]. Free-energy landscapes obtained for each particle orientation can account for configurational entropy, and showed reasonable agreement with results from equivalent multi-site MD simulations ()). It is also possible to develop tractable analytic expressions, especially for some specific geometries. Bianchi et al. [Citation112] derived such an expression for two triblock IPC particles in 3D using point charges located at the centre-of-mass of each of the three charged areas, following Gauss’s law. This method has been adapted for one-patch IPCs [Citation129] by using Poisson’s equation while exploiting superposition. Under conditions of high screening and uniform permittivity, both of these analytic results reduce to a Yukawa-like potential multiplied by an angular dependence, and these reduced forms may be suitable for computational simulations. Elsewhere, studies of the Casimir forces between two JPs in a critical binary liquid mixture have yielded exact expressions for the force [Citation130,Citation131], and more widely-applicable scaling functions for forces and torques within the Derjaguin approximation [Citation132].
3. Kinetics and hydrodynamics
3.1. Kinetic stability and configurational entropy
Kinetically stabilised and non-equilibrium structures are important for JPs because they add possibilities for assembly applications, including hierarchical strategies [Citation50,Citation52,Citation59,Citation121,Citation133,Citation134]. However, there is limited quantitative data available from experiments; simulations of JP clustering have been mostly concerned with identifying low-energy phases and configurations. Kinetically (meta-)stable JP structures readily arise for strong attractive forces [Citation4,Citation123], producing disordered versions of equilibrium structures [Citation135] that (as for isotropic colloids) may be ‘open’, or gel-like. In combination with patch designs, strong attraction enables ‘locking’ [Citation63] and similar assembly strategies.
Weaker attractive forces give rise to interactions between colloids known as ‘floppy’ (or ‘flexible’) bonds. These bonds can break and reform so that configurations that do not minimise the system’s internal energy are thermally explored, without overcoming the overall attractive force. These non-equilibrium dynamics are important for JPs because they produce a wider range of distinct, energetically similar structures than isotropic particles. In an illustrative example () [Citation107]), MD simulations of a pair of hemispherical LJ Janus spheres produced a range of polar angles (θi, θj), but configurations near 55∘ are most frequent. The enthalpy varies very little for ; the probability distribution is explained by configurational (or orientational) entropy – the number of configurations is proportional to
. Entropy strongly affects the collective behaviour of isotropic colloids [Citation33,Citation136], let alone asymmetric particles [Citation137]. Floppy bonds can be regarded as representing free energy equilibrium, so that JP assemblies may be underpinned by entropically stabilised particle orientations.
The range of possible floppy bond angles is sterically limited by the attractive patch size and by the number of binding particles per patch [Citation58,Citation59,Citation99,Citation137]. Control of these factors allows the range of possible bond orientations to be specified [Citation58,Citation137]. When the number of JPs per patch is maximised, entropy contributes to the relative stability of structures [Citation137,Citation138]. Systems with fewer binding colloids per patch are important to study because they produce a wider range of possible configurations, because they produce intermediate [Citation99,Citation139] and open structures (see below), and because they provide an opportunity for better fundamental understanding of interactions.
Various studies have addressed the consequences of floppy bonds for JP assembly. Sciortino’s review [Citation137] includes examples of competition between energy and configurational entropy for patchy and Janus particles. Using KF potentials, configurational entropy has explained an anomalous negatively sloped phase boundary in MC simulations [Citation89], as well as the stability of liquid-like phases at temperatures approaching zero in event-driven MD simulations [Citation140]. MC and BD simulations for triblock particles have suggested that weak interactions are important in hierarchical designs, in order to avoid kinetic trapping in undesired structures [Citation52]. Elsewhere, it has been found that Janus and patchy particles can form six co-ordination elongated helices [Citation139]. This is a so-called open structure that is stabilised by configurational entropy and is unstable for isotropic colloids [Citation138]. Bilayer crystals, another open structure, were consistently observed in another study [Citation95]. Studies of 2D JP assemblies (including theoretical work [Citation138,Citation141]) have played an important role in understanding open structures. In particular, the Kagome lattice formed by triblock colloids [Citation13] is entropically favoured over the isoenergetic close-packed structure () [Citation138]. Other 2D studies have found that high entropy (low co-ordination) configurations are more stable when interparticle bonds are weak [Citation88] and that configurational entropy produces a continuous transition between observed clustering states with decreasing inter-patch attraction [Citation135].
Figure 4. (a) Diagrams of floppy bonds for triblock JPs in 2D. Any ‘bond angle’ is allowed if patches are in contact (top). The hexagonal close packed (middle) and the open Kagome lattice (bottom) structures are isoenergetic, and both have 120∘ bond angles. Reproduced with permission from [Citation138]. (b) Critical shear rate required to break up a single cluster for three interactions strength values C, and for an isotropic hydrophobic case, . Reproduced with permission from [Citation102], conveyed through Copyright Clearance Center, Inc. (c) Kinked Janus clusters with different chirality emerge in a shear flow. Adapted with permission from [Citation97], copyright (2016) American Chemical Society. (d) Simulated JP structures projected onto the plane of a shear gradient, with colouring indicating the size of each cluster. The shear increases with Peclet number (Pe) from left to right. Reproduced with permission from [Citation98], conveyed through Copyright Clearance Center, Inc.
![Figure 4. (a) Diagrams of floppy bonds for triblock JPs in 2D. Any ‘bond angle’ is allowed if patches are in contact (top). The hexagonal close packed (middle) and the open Kagome lattice (bottom) structures are isoenergetic, and both have 120∘ bond angles. Reproduced with permission from [Citation138]. (b) Critical shear rate required to break up a single cluster for three interactions strength values C, and for an isotropic hydrophobic case, Ciso=4. Reproduced with permission from [Citation102], conveyed through Copyright Clearance Center, Inc. (c) Kinked Janus clusters with different chirality emerge in a shear flow. Adapted with permission from [Citation97], copyright (2016) American Chemical Society. (d) Simulated JP structures projected onto the plane of a shear gradient, with colouring indicating the size of each cluster. The shear increases with Peclet number (Pe) from left to right. Reproduced with permission from [Citation98], conveyed through Copyright Clearance Center, Inc.](/cms/asset/c2ab6635-bba3-4293-8cfa-7a8121490354/tapx_a_2341759_f0004_oc.jpg)
3.2. Hydrodynamics and confinement
In experiments, the journey of colloids from fabrication, into solutions, and into clusters inevitably leads to the influence of hydrodynamics. Diffusion dynamics can be significant, and shear arising from applied flows, drainage between particles, or motion of nearby surfaces can provide kinetic stabilisation of aggregates. Therefore, flows and wall-interactions are tools that can be used to engineer assemblies [Citation100]. The general topic of colloids subjected to confinement and shear has previously been reviewed [Citation33]. Despite their importance, hydrodynamics have often been neglected in JP models [Citation142] due to complexity – these forces are inherently long-ranged and many-body in nature.
Relevant studies that have been carried out for JP clusters and aggregates have usually adapted the simulation methods described above. Baran et al.’s [Citation85] MC simulations used hard sphere and square well potentials for hemispherical JPs narrowly confined between walls (4–6 particle diameters apart) in implicit solvent. Observed phases included ordered 2D arrays of small clusters, slabs, and wall-adsorption, in contrast to the large compact aggregates formed without confinement. These researchers have also studied confined 2D Janus disks [Citation143], and the effect of JP density on wall adsorption [Citation101].
In MD simulations, a Yukawa-type pair interaction model was used for confined amphiphilic Janus spheres assembling in shear flow [Citation102]. Micelles which formed at low shear rates broke up into smaller clusters when sufficiently strong shear was applied (). Huang et al. [Citation97] used MD simulations with an LJ pair potential and the MPCD method, a combination which allowed inclusion of explicit solvent. This study also identified both shear-enhanced clustering and disaggregation at greater shear. Cluster bonding arrangements affected the response to shear (e.g. there were transitions between clusters of different chirality, ), and the energy associated with shear-induced disassembly was analysed.
Multi-site Janus spheres () have been studied in a shear flow imposed via a reverse perturbation method, without walls, and aided by use of the MPCD approach to include explicit solvent [Citation144]. At rest, JPs with small hydrophobic patches formed spherical micelles, whereas hemispherical JPs aggregated into worm-like structures. With applied shear, the micelles deformed and rearranged, then at high enough shear broke up into dimers and single particles. Similar methods were used to simulate mixtures of JPs with polymer chains [Citation109]. Another study that imposed shear via the reverse perturbation method [Citation145] simulated rigid LJ trimer assemblies with implicit solvent. Extended, globular micelles were produced, and their size increased with shear. Shear can also be imposed in BD simulations with implicit solvent using Lees – Edwards boundary conditions, where the velocity of particles crossing the simulation boundary in one direction is adjusted according to a specified steady shear rate [Citation97,Citation98]. In such a study [Citation98], micelles and vesicles slightly increased in size when small shear was imposed (Pe = 1 in )). At higher shear rates, worm-like micelles broke up into intermediate elongated micelles.
Simulations of the effect of shear on JP aggregation have therefore produced some consistent trends. When spherical or globular micelles are present at rest, shear produces deformed and elongated shapes [Citation144,Citation145]. At low shear rates, micelles can be encouraged to form or increase in size [Citation97,Citation98,Citation100,Citation102,Citation145], while high shear leads to break-up into smaller micelles, and ultimately dimers and single JPs [Citation97,Citation98,Citation100,Citation144], with dependence on the interaction strength () [Citation102]). In some studies, clusters have been particularly affected by confinement or nearby walls [Citation85,Citation100,Citation101].
Explicit modelling of solvent particles, which is likely to be important to accurately capture hydrodynamic effects, has notably been enabled by the efficiency of the MPCD method for many JPs [Citation100,Citation144]. For one or two JPs, MD studies of multi-site particles within explicitly modelled solvent particles has allowed more detailed studies of hydrodynamics. Safaei et al. have used their multi-site model ()) to study slip boundary conditions on JP surfaces, including the slip-induced dynamics (force and torque) in a uniform flow [Citation117]. Pairs of JPs were then studied [Citation118], firstly to establish the thermal break-up rate, then the stability of a dimer within a shear flow induced by moving non-slip walls [Citation118]. Uniform hydrophobic dimers were more stable than Janus dimers, while dimer stability appeared to have some dependence on the slip length (relative hydrophobicity) of the JPs. In these studies the slip lengths were estimated by studying flows induced between flat walls similar to the JP surfaces, a method also used by Kharazmi et al. [Citation108], who found that translational and rotational diffusion coefficients increase with the hydrophobicity of the non-wetting hemisphere on a JP. Another study featuring amphiphilic multi-site Janus spheres sheared by explicit solvent used dissipative particle dynamics, and confined the JPs within nanotubes [Citation125]. In later simulations, shear flow was imposed by non-slip walls [Citation80]. In a study which coupled MD for multi-site JPs (radius 4σ) with lattice Boltzmann simulations of the solvent [Citation146], hydrodynamics were included via a viscous friction term. The JPs and ions in solution were governed by WCA and Coulomb potentials, and the effect of an applied electric field was studied.
Among other hydrodynamic simulations, Kohl et al. [Citation142] recently numerically modelled up to 150 interacting Janus spheres. This study used boundary integral methods with spherical harmonic expansions to evaluate spectrally accurate integral operators. Interactions were explored for amphiphilic, charged dipole, and phoretic particles, all using a screened electrostatic potential. Elsewhere, 3D hydrodynamics around fixed JPs have been studied using computational fluid dynamics [Citation142,Citation147–149].
4. Experiments
Experimental work involving 3D self-assembly of spherical Janus particles in bulk solution is much less common than theoretical work. For advancing fundamental understanding, the most interesting studies are those in which clustering kinetics have been quantified, and/or experimental structures have been compared with simulations. Perhaps the most influential work has been carried out using experimentally pragmatic ‘amphiphilic’ JPs in which hydrophobic patches are added to charged particles. Such particles remain in stable dispersion due to electrostatic repulsion prior to the addition of salt, which screens the charge and allows clusters to form. For example, carboxylated particles have been modified with a hydrophobic silane on one side [Citation84], and sulphate-functionalised particles were similarly modified with a thiol layer [Citation139]. Results have revealed how the electrostatic interaction range can be tuned by adding salt to obtain different aggregation and phase behaviours. With no salt added, particles remain dispersed. Competition between hydrophobic attraction and electrostatic repulsion arises when interparticle interactions are short-ranged relative to particle size – the JPs form small clusters (short-ranged assemblies) around 1 mM, and worm-like strings and helices with various isomers (longer-ranged assemblies) around 5 mM.
In the first of these studies [Citation84], conversion between isomeric clusters was reportedly observed on time scales of seconds to minutes using fluorescence microscopy (). Simulated MC structures reproduced those observed, including the isomers, when a point potential invoking DLVO theory (Section 2) was used with an attractive strength of 5–10 . The second study [Citation139] more extensively tracked dynamic changes in cluster size and shape, for example, measuring cluster size distributions (n = 1 to 7) over the course of an hour and measuring first-order kinetics for the transition between two non-equilibrium n = 6 isomers. It was found that small, favoured isomers formed and then fused into open, worm-like helices. The forms of these structures were consistent with calculations of free energy that included rotational entropy and could be reproduced in MD simulations using a rounded potential at the patch edge.
Figure 5. (a) Suggested transitions between isomers consisting of 7 fluorescent amphiphilic 1 µm polystyrene JPs (above), consistent with MC-simulated structures (below). Reproduced with permission from [Citation84], copyright (2008) American Chemical Society. (b) Three types of bonding geometries identified between small clusters of 1 µm triblock JPs, along with the measured occurrence probability (P) of these geometries for 4-particle clusters. Adapted with permission from [Citation121], copyright (2012) American Chemical Society. (c) Histogram of kink angles (left) obtained from images of polystyrene ~ 2 µm bipolar JPs (right), scale bar 20 µm. Reproduced with permission from [Citation103], conveyed through Copyright Clearance Center, Inc. (d) Optical images of DNA-patch JPs at two different temperatures (scale bar 10 µm), and the corresponding cluster size distributions where ϵ is the depth of the attractive potential in simulations giving closest agreement. Adapted with permission from [Citation99]. (e) ‘Rafts’ of DNA patches on polymer colloid surfaces selectively bind to their conjugate, allowing chains of alternating colloids to form. Reproduced with permission from [Citation150], copyright 2018 National Academy of Sciences.
![Figure 5. (a) Suggested transitions between isomers consisting of 7 fluorescent amphiphilic 1 µm polystyrene JPs (above), consistent with MC-simulated structures (below). Reproduced with permission from [Citation84], copyright (2008) American Chemical Society. (b) Three types of bonding geometries identified between small clusters of 1 µm triblock JPs, along with the measured occurrence probability (Pi,N) of these geometries for 4-particle clusters. Adapted with permission from [Citation121], copyright (2012) American Chemical Society. (c) Histogram of kink angles (left) obtained from images of polystyrene ~ 2 µm bipolar JPs (right), scale bar 20 µm. Reproduced with permission from [Citation103], conveyed through Copyright Clearance Center, Inc. (d) Optical images of DNA-patch JPs at two different temperatures (scale bar 10 µm), and the corresponding cluster size distributions where ϵ is the depth of the attractive potential in simulations giving closest agreement. Adapted with permission from [Citation99]. (e) ‘Rafts’ of DNA patches on polymer colloid surfaces selectively bind to their conjugate, allowing chains of alternating colloids to form. Reproduced with permission from [Citation150], copyright 2018 National Academy of Sciences.](/cms/asset/f0e78bfe-a7d1-4894-b496-2f9720da85ad/tapx_a_2341759_f0005_oc.jpg)
Research using similar amphiphilic triblock particles has also contributed to both kinetics data and theoretical comparisons [Citation121]. These JPs were made by thiolating gold polar caps on sulphate-functionalised particles, enabling self-assembly that could be staged based on the size of the caps and salt concentration. Small clusters formed during a first stage were joined together by bonding ‘geometries’ (linear, triangular, and square) at a second stage. The statistics of this process were measured, including the distribution of small cluster sizes (n = 4 to 6) that is stable after ~ 20 minutes, the prevalence of the bonding geometries once salt is added (), and the statistics of cluster polymerisation into larger chains over ~ 4 minutes. A calculation of effective patch size, which was broadly consistent with the observed staging, used a pair of particles each having > 8000 sites interacting via a Yukawa potential, and with an estimated attractive interaction strength of ~ 7 kBT based on previous work. A later simulation study [Citation50] also noted the similarity of their configuration to the experiments in [Citation121]. The results of these simulations were shown to tolerate dispersity, which is an important practical consideration due to imperfect fabrication. The same simulation method has been extended to explore programmed design of structures for optical applications [Citation51,Citation52].
In an earlier study, carboxylate-modified (negative) 1 µm polystyrene colloids were modified by depositing a thin gold film and attaching a monolayer to produce positive charge [Citation110]. These ‘zwitterionic’ JPs are therefore similar but not identical to amphiphilic JPs. In 1 mM salt (10 nm) small clusters such as octahedrons were observed via fluorescence and the preferred structures were identified based on the frequency of their occurrence (especially up to n = 7). The experimental clusters were compared with MC simulations, firstly with an interaction based on a numerical model for electrostatic interactions between hemispheres, and secondly with an LJ interaction (see Section 2). Preferred cluster shapes matched the simulations for most (but not all) values of n. The experimental results have also been used to claim that other simulations [Citation126] produce agreement with experiment, at least for clusters of up to 8 particles.
In experiments using similar zwitterionic JPs [Citation103], 2–3 µm positively charged (amidine) polystyrene particles were modified via hydrolysis at an air-water interface to produce IPCs with negative charge on one side. This study investigated the effects of patch coverage, salt and particle concentrations, and isotropic particles on assembly. Conditions producing finite clusters, chains, and irregular amorphous structures were identified. Particles could be observed using optical microscopy, so that data such as particles per chain and the angle between successive particles could be obtained ()). The low occurrence of ‘kink’ angles was found to be consistent with a theoretical potential. Results of a BD study [Citation113] were also compared with these experiments.
JP interactions can also be realised using DNA patches, and Oh et al. [Citation99] have observed floppy bonding on JPs with mutually attractive patches featuring DNA strands bound to polystyrene. An emulsion polymerisation fabrication method was used to closely control patch sizes on the otherwise repulsive polymer particles. These JPs were dispersed in a non-stick capillary tube and thermally reversible cluster formation was observed as they were held at raised temperatures for up to a day. For relatively small patches, the occurrence of small n = 1 to 4 clusters was measured ()), with patch connection confirmed using fluorescence microscopy. MD simulations were carried out using a Morse potential for attractive interactions between DNA patches and a WCA potential acting between non-patch regions, with some angular modulation, and with depletion interactions included via an extra LJ potential. The simulations indicated broad agreement between simulated and observed cluster sizes, while the best-fitting values of / ϵ suggested that temperature-induced changes were due to variation in the DNA interactions, rather than Brownian effects.
JPs with larger DNA patches [Citation99] could sterically accommodate > 3 bonds per particle so that clusters of n = 4 to 7 particles were observed joining together into larger-scale structures which depended on patch size rather than temperature: flexible dimer chains, trimer stacks, and hexagonally packed bilayers. After aggregate formation, these structures reoriented towards equilibrium configurations identified in simulations. It was noted that Chen et al. [Citation139] may have in fact observed equilibrium (rather than open) structures due to non-uniform particle coating, a fabrication effect that adds orientation dependence to particle interactions. More recently, Oh et al. [Citation151] have extended their experimental observations over a wider range of temperatures, patch sizes, and surface chemistries, while also using improved fluorescence imaging, which may allow observations of JP orientation within structures.
The experiments mentioned above have provided the main quantitative evidence regarding 3D spherical JP clustering in bulk fluid. They have employed various fabrication and simulation methods so that it is difficult to identify universal trends, but a broad summary is possible. Single-patch JP aggregates appear to range from small clusters, to worm-like chains, and to bilayers, with dependence mostly on patch size [Citation99]. Larger-scale structures appear to form from smaller clusters of n ≈6 [Citation99,Citation139]. Structural changes to clusters are observed, and appear to produce structures that are consistent with calculated equilibrium structures. Measurements of clustering kinetics have been reported [Citation121,Citation139] but have not been accompanied by strong theoretical descriptions. There have been several comparisons of cluster structures between experiments and simulations [Citation84,Citation110,Citation121]. Broad validations of the models have been attempted by considering interaction potential strengths for particular materials [Citation84,Citation99], but inconsistencies remain. For example, a small interaction range is predicted to produce more open and less ordered structures [Citation90] than the close-packed clusters and helices observed [Citation84,Citation139], and indeed van der Waals forces should cause JPs to aggregate irreversibly at very high salt concentrations [Citation152]. There remain questions about clustering dynamics, the role of orientational entropy, and the associated competition between Brownian motion and interaction strength. Measurement of particle orientation has been an important omission in these studies.
A range of other experiments have involved less quantitative analysis, or do not involve spherical JPs in bulk, but may still be instructive. For example, Tsyrenova et al. [Citation153] have studied the effect of various surfactants on assembly of 3 µm amphiphilic JPs, presenting measurements describing the fraction of JPs within clusters, and the structure and rotational dynamics of the JPs after formation of 2D colloidal crystals on a substrate. This study suggested that van der Waals forces (along with hydrophobic interactions) were important in the assembly process. Elsewhere, DNA-modified JPs have been used to produce strong attractive interactions that cause particles to irreversibly aggregate according to patch geometry, without exploring configurations as in floppy bonds. Systems involving interactions between complementary nucleic acids (‘lock and key colloids’ [Citation154]) demonstrate these concepts. For example, self-assembly can be guided by patterning ssDNA asymmetrically on a spherical particle [Citation155,Citation156]. Recent examples include using DNA to encode assembly of novel 3D colloidal crystal architectures [Citation157], and alternating chains via DNA rafts ()) [Citation150].
For nanoscale JP clustering, characterisation typically requires sample preparation steps to observe end-products initially formed in a bulk 3D solution. For example, Groschel et al. [Citation158] observed chains and slightly larger networks formed by near-spherical 50 nm triblock patchy particles built from ~ 10 nm terpolymers. Aggregate structures were visualised using TEM after drop-casting on a substrate, and the triblock orientation was confirmed. Structure growth could be controlled using e.g. laterally exposed and end-capping units. Significantly aspherical JPs such as these reduce the possibility of validating theories which assume that the JPs are spherical. For example, experimentally observed dendrimers formed by non-spherical JPs have been compared with simulations that used spherical JPs [Citation81]. Kang et al. [Citation16,Citation159] were able to study how ‘snowman’ geometries influenced assemblies, making comparisons with structures assembled using triblock JPs [Citation160]. In this case, the orientations of the assembled particles could be observed but the dynamics of how the clusters formed is unclear. Finally, the analogy between non-attractive patches and repulsion due to steric hindrance from grafted polymers has been quantitatively explored, demonstrating some equivalence between these approaches [Citation161].
Future experimental studies of 3D JP interactions in bulk fluid can also take some inspiration from previous work involving 2D assemblies on surfaces and at interfaces. For example, the practical possibility of floppy bonds giving rise to entropically stabilised open structures has been most clearly demonstrated by studies of Kagome lattices [Citation13,Citation138]. Imaging of individual particle orientations, and comparisons with modelling, have been achieved using 3 µm JPs [Citation162] which cluster during drying ()), using 1–2 µm close-packed JPs [Citation135,Citation163] ()), and using 300 nm amphiphilic JPs observed using SEM [Citation119]. JP monolayers can also assemble at a fluid-fluid interface, in which case capillary forces are usually dominant over other interparticle interactions [Citation162,Citation166] and the orientations of relatively large JPs (e.g. 100–200 µm [Citation167]) can be observed and analysed.
Figure 6. Experimental visualisations of JP orientations. (a) Clusters formed via a drying process from 3 µm silica JPs with Au-coated thiolated hemispheres (scale bar 10 µm) along with statistics for the out-of-plane angle θ. Reproduced with permission from [Citation162], conveyed through Copyright Clearance Center, Inc. (b) 2D visualisation of 1.5 µm silica JPs, with scale bars 10 µm (main image) and 2 µm (inset). Reproduced with permission from [Citation163], conveyed through Copyright Clearance Center, Inc. (c) Time sequence showing changes in orientation for two approaching Ni-coated 3 µm silica JPs in a precessing magnetic field, with scale bar 2 µm. Reproduced with permission from [Citation164]. (d) Au-coated 20 µm fluorescent latex JPs in an electric field, with scale bar 2 µm. The field polarity is reversed between the top and bottom images. Reproduced with permission from [Citation165], copyright (1997) American Chemical Society.
![Figure 6. Experimental visualisations of JP orientations. (a) Clusters formed via a drying process from 3 µm silica JPs with Au-coated thiolated hemispheres (scale bar 10 µm) along with statistics for the out-of-plane angle θ. Reproduced with permission from [Citation162], conveyed through Copyright Clearance Center, Inc. (b) 2D visualisation of 1.5 µm silica JPs, with scale bars 10 µm (main image) and 2 µm (inset). Reproduced with permission from [Citation163], conveyed through Copyright Clearance Center, Inc. (c) Time sequence showing changes in orientation for two approaching Ni-coated 3 µm silica JPs in a precessing magnetic field, with scale bar 2 µm. Reproduced with permission from [Citation164]. (d) Au-coated 20 µm fluorescent latex JPs in an electric field, with scale bar 2 µm. The field polarity is reversed between the top and bottom images. Reproduced with permission from [Citation165], copyright (1997) American Chemical Society.](/cms/asset/bba31382-9fd3-4ae5-8af9-41355b2bac68/tapx_a_2341759_f0006_oc.jpg)
5. Discussion and conclusions
Opportunities for future research into 3D assemblies of Janus spheres will undoubtedly include refinement of the potentials acting between JPs in simulations [Citation73]. Stable structures can be highly sensitive to details of the interaction [Citation95], and at least one study has noted that point potentials may be inadequate [Citation126]. The asymmetry of potentials deserves particular attention. Angular functions used with point potentials have included sharp transitions, a continuously (e.g. sinusoidally) varying interaction [Citation50,Citation52,Citation81,Citation105], or a smoothing function [Citation83,Citation97,Citation98] ()). These forms are mostly arbitrary and may be oversimplified [Citation77], although a sharp transition can be justified when so that repulsion is limited to a small area near the point of contact, and short interaction lengths (e.g. λ = 1.05) are experimentally realised for micrometre-scale JPs when λD or the hydrophobic force typically act over ~ 10 nm [Citation71,Citation84]. Multi-site, analytic and numerical approaches can derive more physically justifiable potentials at greater computational expense, and point potentials which approximate more detailed calculations have been used to enable larger-scale simulations [Citation110,Citation126].
It is also fair to say that a wide range of simulations has already been reported so that the present challenge is tailoring of interactions to provide greater insight into physically realistic experimental systems. A small range of MC, MD, BD, and numerical methods have been used for direct comparisons with experiments, as described in Section 4. Simulations have tended not to engage with questions about the fundamental nature of hydrophobicity [Citation84], while experimental collections of JPs are very unlikely to be uniform in size (an important topic for isotropic colloids [Citation136]), patch geometry, and chemistry. Variability can arise from artefacts of fabrication [Citation99], patch engineering [Citation4], and the combination of these factors with non-spherical particles. There are also myriad different types of JP surfaces and solutions to explore, along with the sizes of JPs and patches relative to the interaction length scale.
The importance of non-equilibrium and kinetic effects suggests enormous opportunity to study both fundamental behaviours and to develop assembly strategies for future technologies. For floppy bonds, the interplay between co-ordination, patch size, and patch position provides a huge set of novel structural possibilities. Hydrodynamic simulations have addressed diffusion [Citation108], interactions with walls and interfaces [Citation85,Citation119,Citation120,Citation125], and dynamics in imposed flows [Citation117,Citation125]. Janus dimers have been studied interacting under thermal motion [Citation107] and breaking apart in shear flow [Citation118]. Multiple-particle clustering has also been studied, aided by efficiency of the MPCD approach for modelling explicit solvent [Citation109,Citation144]. Hydrodynamics will play a role in virtually any practical experiment or assembly process and clearly ongoing JP research ‘should include the addition of hydrodynamic interactions’ [Citation98]. Inspiration may be found in studies of isotropic colloids, and in fields such as self-propelling active matter, which has produced strong hydrodynamic studies [Citation75]. 3D simulations and theoretical methods exploring the parameter space should account for configurational entropy either explicitly, or implicitly such as when MD simulations are used to record the distribution of accessed configurations [Citation107] ()). Following paradigms for isotropic colloids, there is room for more theoretical research based on thermodynamics and statistical mechanics [Citation141], and statistical theories may describe structure relaxation in response to parameters such as shear (characterised by a Peclet number) and cooling rates.
A corollary of the opportunities above is that there is a recognised [Citation4,Citation113], pressing need for more, and more well-controlled, quantitative experimental data. Highly controlled fabrication [Citation99] and precise experimental configurations are required to test and validate theoretical approaches [Citation141], and the use of controlled experiments (including observations of dynamics) is clearly relevant for practical technological developments. Some of the most interesting possibilities for JPs rely on weak attractive forces (such as van der Waals) in competition with Brownian motion, or on interesting non-equilibrium and dynamic effects, but it is difficult to construct controlled assemblies in these cases [Citation92]. The initial conditions for JP experiments are seldom discussed but are essential for practical and quantitative studies. These conditions include the spatial distribution of JPs when observations commence, and their prior opportunity to interact. Controlling these parameters requires close characterisation of how the JPs are fabricated, stored, concentrated and diluted, and allowed to diffuse.
Section 4 described how experiments to date have provided some insights into clustering by comparing observed and simulated structures, with some consideration of statistics and kinetics, but they have also raised new questions. A recurring limitation of experimental 3D clustering studies is that orientations of individual JPs have usually been inferred from simulations, rather than directly measured. In particular, there is demand for quantitative, systematic and controlled measurements, and it would be good to obtain time-resolved data during aggregation. These data would allow closer validation of important theoretical aspects such as the orientational dependence of interactions, and dynamics arising from diffusion, hydrodynamics, and chemical interactions. Image analysis tools for this task have been developed [Citation168–170], although stereoscopic imaging would be necessary for fully 3D analysis. Brownian motion plays an important role in many of the most interesting systems, so the size of the colloids presents practical challenges for imaging. Examples of clear optical visualisation experiments are available from some 2D studies (e.g. ) [Citation162,Citation163]), while particle dynamics have been recorded for relatively large JPs responding to applied fields in bulk fluid [Citation31,Citation59,Citation171]. ) shows magnetic Janus spheres dynamically forming a dimer, with their orientation depending on an applied field and induced dipole-dipole interactions [Citation172–175]. These JPs can aggregate further to form orientation-resolved microtubes [Citation164]. ) is a classic observation of 20 µm JPs rotating according to the direction of an applied electric field [Citation165], which is caused by polarisation of conductive materials [Citation176,Citation177].
Two further emerging research directions deserve to be pointed out. Firstly, indirect experimental methods can be used characterise JP interaction potentials. Colloidal probe atomic force microscopy has been used to measure forces as a function of orientation near an air-water interface for amphiphilic JPs [Citation178]. This technique can be extremely sensitive so that gathering more extensive and precise data should be possible. Secondly, machine learning methods can be applied to colloidal structures [Citation179]. In one example, a neural network approach has been applied to identify and classify local and disordered systems governed by the LJ potential [Citation112]. A related idea is so-called reverse engineering of patchy particles, where machine learning is used to produce a target aggregate (e.g. a hollow capsid [Citation180] or a photonic crystal [Citation181]) by navigating energy landscapes.
For assembly applications, underlying concepts have been established and design rules have been discussed but there remains lack of detail in the theoretical and experimental understanding which will ultimately underpin the field. It is likely that ‘self-assembler’ technologies [Citation33] will need to be developed. Both technologies and fundamental studies may benefit by using a combination of controlled hydrodynamics (especially via microfluidics) with applied magnetic, electric, or optical forces. These endeavours will draw researchers from a wide range of disciplines, with experimental, theoretical, and computational physicists likely to be prominent among them.
Disclosure statement
No potential conflict of interest was reported by the author(s).
Funding
This work was supported by the MacDiarmid Institute for Advanced Materials and Nanotechnology.
Additional information
Funding
References
- Hughes LJ, Brown GL. Heterogeneous polymer systems. I. Torsional modulus studies. J Appl Polym Sci. 1961;5:580–588. doi: 10.1002/app.1961.070051713
- Casagrande C, Fabre P, Raphaël E, et al. “Janus beads”: realization and behaviour at water/oil interfaces. Europhys Lett (EPL). 1989;9:251–255. doi: 10.1209/0295-5075/9/3/011
- De Gennes PG. Soft matter (nobel lecture). Angew Chem Int Ed Engl. 1992;31:842–845. doi: 10.1002/anie.199208421
- Duguet E, Hubert C, Chomette C, et al. Patchy colloidal particles for programmed self-assembly. C R Chim. 2016;19:173–182. doi: 10.1016/j.crci.2015.11.013
- Maula TA, Hatch HW, Shen VK, et al. Designing molecular building blocks for the self-assembly of complex porous networks. Mol Syst Des Eng. 2019;4:644–653. doi: 10.1039/C9ME00006B
- Noya EG, Zubieta I, Pine DJ, et al. Assembly of clathrates from tetrahedral patchy colloids with narrow patches. J Chem Phys. 2019;151:094502. doi: 10.1063/1.5109382
- Glotzer SC, Solomon MJ. Anisotropy of building blocks and their assembly into complex structures. Nature Mater. 2007;6:557–562. doi: 10.1038/nmat1949
- Hou W, Zhong W, Zhao H. Asymmetric colloidal particles fabricated by polymerization-induced surface self-assembly approach. Macromolecules. 2021;54:2617–2626. doi: 10.1021/acs.macromol.0c02772
- Pradhan SS, Saha S. Advances in design and applications of polymer brush modified anisotropic particles. Adv Colloid Interface Sci. 2022;300:102580. doi: 10.1016/j.cis.2021.102580
- Cayre O, Paunov VN, Velev OD. Fabrication of asymmetrically coated colloid particles by microcontact printing techniques. J Mater Chem. 2003;13:2445. doi: 10.1039/b308817k
- Nisisako T, Torii T, Takahashi T, et al. Synthesis of monodisperse bicolored Janus particles with electrical anisotropy using a microfluidic co-flow system. Adv Mater. 2006;18:1152–1156. doi: 10.1002/adma.200502431
- Zhang G, Wang D, Möhwald H. Decoration of microspheres with gold nanodots—giving colloidal spheres valences. Angewandte Chemie. 2005;44:7767–7770. doi: 10.1002/anie.200502117
- Chen Q, Bae SC, Granick S. Directed self-assembly of a colloidal kagome lattice. Nature. 2011;469:381–384. doi: 10.1038/nature09713
- Jackson AM, Hu Y, Silva PJ, et al. From homoligand- to mixed-ligand- monolayer-protected metal nanoparticles: a scanning tunneling microscopy investigation. J Am Chem Soc. 2006;128:11135–11149. doi: 10.1021/ja061545h
- Liu B, Liu J, Liang F, et al. Robust anisotropic composite particles with tunable Janus balance. Macromolecules. 2012;45:5176–5184. doi: 10.1021/ma300409r
- Kang C, Honciuc A. Influence of geometries on the assembly of snowman-shaped Janus nanoparticles. ACS Nano. 2018;12:3741–3750. doi: 10.1021/acsnano.8b00960
- Deng R, Liang F, Zhou P, et al. Janus nanodisc of diblock copolymers. Adv Mater. 2014;26:4469–4472. doi: 10.1002/adma.201305849
- Chen Y, Liang F, Yang H, et al. Janus nanosheets of polymer–inorganic layered composites. Macromolecules. 2012;45:1460–1467. doi: 10.1021/ma2021908
- Chaudhary K, Chen Q, Juárez JJ, et al. Janus colloidal matchsticks. J Am Chem Soc. 2012;134:12901–12903. doi: 10.1021/ja305067g
- Yan J, Chaudhary K, Chul Bae S, et al. Colloidal ribbons and rings from Janus magnetic rods. Nat Commun. 2013;4:1516. doi: 10.1038/ncomms2520
- Kim JW, Larsen RJ, Weitz DA. Synthesis of nonspherical colloidal particles with anisotropic properties. J Am Chem Soc. 2006;128:14374–14377. doi: 10.1021/ja065032m
- Ma X, Jannasch A, Albrecht UR, et al. Enzyme-powered hollow mesoporous Janus nanomotors. Nano Lett. 2015;15:7043–7050. doi: 10.1021/acs.nanolett.5b03100
- Han YD, Kim HS, Park YM, et al. Retroreflective Janus microparticle as a nonspectroscopic optical immunosensing probe. ACS Appl Mater Inter. 2016;8:10767–10774. doi: 10.1021/acsami.6b02014
- Li W, Palis H, Mérindol R, et al. Colloidal molecules and patchy particles: complementary concepts, synthesis and self-assembly. Chem Soc Rev. 2020;49:1955–1976. doi: 10.1039/C9CS00804G
- Frank BD, Perovic M, Djalali S, et al. Synthesis of polymer Janus particles with tunable wettability profiles as potent solid surfactants to promote gas delivery in aqueous reaction media. ACS Appl Mater Inter. 2021;13:32510–32519. doi: 10.1021/acsami.1c07259
- Bianchi E, Van Oostrum PD, Likos CN, et al. Inverse patchy colloids: Synthesis, modeling and self-organization. Curr Opin Colloid Interface Sci. 2017;30:8–15. doi: 10.1016/j.cocis.2017.03.010
- Li X, Chen L, Cui D, et al. Preparation and application of Janus nanoparticles: Recent development and prospects. Coord Chem Rev. 2022;454:214318. doi: 10.1016/j.ccr.2021.214318
- Zhang J, Grzybowski BA, Granick S. Janus particle synthesis, assembly, and application. Langmuir. 2017;33:6964–6977. doi: 10.1021/acs.langmuir.7b01123
- Pang X, Wan C, Wang M, et al. Strictly biphasic soft and hard Janus structures: synthesis, properties, and applications. Angewandte Chemie. 2014;53:5524–5538. doi: 10.1002/anie.201309352
- Poggi E, Gohy JF. Janus particles: from synthesis to application. Colloid Polym Sci. 2017;295:2083–2108. doi: 10.1007/s00396-017-4192-8
- Walther A, Müller AHE. Janus particles: synthesis, self-assembly, physical properties, and applications. Chem Rev. 2013;113:5194–5261. doi: 10.1021/cr300089t
- Lattuada M, Hatton TA. Synthesis, properties and applications of Janus nanoparticles. Nano Today. 2011;6:286–308. doi: 10.1016/j.nantod.2011.04.008
- Krishnamurthy S, Mathews Kalapurakal RA, Mani E. Computer simulations of self-assembly of anisotropic colloids. J Phys. 2022;34:273001. doi: 10.1088/1361-648X/ac55d6
- Gheisari F, Shafiee M, Abbasi M, et al. Janus nanoparticles: an efficient intelligent modern nanostructure for eradicating cancer. Drug Metab Rev. 2021;53:592–603. doi: 10.1080/03602532.2021.1878530
- Li Z, Gao Z, Wang C, et al. Recent progress on bioimaging strategies based on Janus nanoparticles. Nanoscale. 2022;14:12560–12568. doi: 10.1039/D2NR03186H
- He Q, Vijayamohanan H, Li J, et al. Multifunctional photonic Janus particles. J Am Chem Soc. 2022;144:5661–5667. doi: 10.1021/jacs.2c01787
- Campuzano S, Gamella M, Serafín V, et al. Magnetic Janus particles for static and dynamic (bio)sensing. Magnetochemistry. 2019;5:47. doi: 10.3390/magnetochemistry5030047
- Su H, Hurd Price CA, Jing L, et al. Janus particles: design, preparation, and biomedical applications. Mater Today Bio. 2019;4:100033. doi: 10.1016/j.mtbio.2019.100033
- Patra D, Sengupta S, Duan W, et al. Intelligent, self-powered, drug delivery systems. Nanoscale. 2013;5:1273–1283. doi: 10.1039/C2NR32600K
- Tan KX, Danquah MK, Jeevanandam J, et al. Development of Janus particles as potential drug delivery systems for diabetes treatment and antimicrobial applications. Pharmaceutics. 2023;15:423. doi: 10.3390/pharmaceutics15020423
- Shaghaghi B, Khoee S, Bonakdar S. Preparation of multifunctional Janus nanoparticles on the basis of SPIONs as targeted drug delivery system. Int J Pharmaceut. 2019;559:1–12. doi: 10.1016/j.ijpharm.2019.01.020
- Marschelke C, Fery A, Synytska A. Janus particles: from concepts to environmentally friendly materials and sustainable applications. Colloid Polym Sci. 2020;298:841–865. doi: 10.1007/s00396-020-04601-y
- Cao W, Huang R, Qi W, et al. Self-assembly of amphiphilic Janus particles into monolayer capsules for enhanced enzyme catalysis in organic media. ACS Appl Mater Inter. 2015;7:465–473. doi: 10.1021/am5065156
- Kirillova A, Schliebe C, Stoychev G, et al. Hybrid hairy Janus particles decorated with metallic nanoparticles for catalytic applications. ACS Appl Mater Inter. 2015;7:21218–21225. doi: 10.1021/acsami.5b05224
- Chen C, Zhang L, Wang N, et al. Janus composite particles and interfacial catalysis thereby. Macromol Rapid Commun. 2023;44:2300280. doi: 10.1002/marc.202300280
- Vafaeezadeh M, Thiel WR. Task-specific Janus materials in heterogeneous catalysis. Angewandte Chemie. 2022;61:e202206403. doi: 10.1002/anie.202206403
- McConnell MD, Kraeutler MJ, Yang S, et al. Patchy and multiregion Janus particles with tunable optical properties. Nano Lett. 2010;10:603–609. doi: 10.1021/nl903636r
- Minin OV, Zhou S, Liu CY, et al. Magnetic concentric hot-circle generation at optical frequencies in all-dielectric mesoscale Janus particles. Nanomaterials. 2022;12:3428. doi: 10.3390/nano12193428
- Trivedi M, Saxena D, Ng WK, et al. Self-organized lasers from reconfigurable colloidal assemblies. Nat Phys. 2022;18:939–944. doi: 10.1038/s41567-022-01656-2
- Morphew D, Shaw J, Avins C, et al. Programming hierarchical self-assembly of patchy particles into colloidal crystals via colloidal molecules. ACS Nano. 2018;12:2355–2364. doi: 10.1021/acsnano.7b07633
- Neophytou A, Manoharan VN, Chakrabarti D. Self-assembly of patchy colloidal rods into photonic crystals robust to stacking faults. ACS Nano. 2021;15:2668–2678. doi: 10.1021/acsnano.0c07824
- Rao AB, Shaw J, Neophytou A, et al. Leveraging hierarchical self-assembly pathways for realizing colloidal photonic crystals. ACS Nano. 2020;14:5348–5359. doi: 10.1021/acsnano.9b07849
- Flavell W, Neophytou A, Demetriadou A, et al. Programmed self-assembly of single colloidal gyroids for chiral photonic crystals. Adv Mater. 2023;35:2211197. doi: 10.1002/adma.202211197
- Correia EL, Brown N, Razavi S. Janus particles at fluid interfaces: stability and interfacial rheology. Nanomaterials. 2021;11:374. doi: 10.3390/nano11020374
- Qiao Y, Ma X, Liu Z, et al. Tuning the rheology and microstructure of particle-laden fluid interfaces with Janus particles. J Colloid Interface Sci. 2022;618:241–247. doi: 10.1016/j.jcis.2022.03.041
- Walther A, Hoffmann M, Müller A. Emulsion polymerization using Janus particles as stabilizers. Angew Chem. 2008;120:723–726. doi: 10.1002/ange.200703224
- Sun D, Si Y, Song XM, et al. Bi-continuous emulsion using Janus particles. Chem Comm. 2019;55:4667–4670. doi: 10.1039/C9CC01191A
- Wang Y, Wang Y, Breed DR, et al. Colloids with valence and specific directional bonding. Nature. 2012;491:51–55. doi: 10.1038/nature11564
- Zhang J, Luijten E, Granick S. Toward design rules of directional Janus colloidal assembly. Annu Rev Phys Chem. 2015;66:581–600. doi: 10.1146/annurev-physchem-040214-121241
- Yammine E, Souaid E, Youssef S, et al. Particles with magnetic patches: synthesis, morphology control, and assembly. Part Part Syst Charact. 2020;37:2000111. doi: 10.1002/ppsc.202000111
- Pelesko JA. Self assembly: the science of things that put themselves together. London: Chapman and Hall/CRC; 2007.
- Glotzer SC, Solomon MJ, Kotov NA. Self-assembly: from nanoscale to microscale colloids. AichE J. 2004;50:2978–2985. doi: 10.1002/aic.10413
- Chen Q, Yan J, Zhang J, et al. Janus and multiblock colloidal particles. Langmuir. 2012;28:13555–13561. doi: 10.1021/la302226w
- Yu B, Cong H, Peng Q, et al. Current status and future developments in preparation and application of nonspherical polymer particles. Adv Colloid Interface Sci. 2018;256:126–151. doi: 10.1016/j.cis.2018.04.010
- Patra TK, Katiyar P, Singh JK. Substrate directed self-assembly of anisotropic nanoparticles. Chem Eng Sci. 2015;121:16–22. doi: 10.1016/j.ces.2014.09.023
- Liddle JA, Gallatin GM. Nanomanufacturing: a perspective. ACS Nano. 2016;10:2995–3014. doi: 10.1021/acsnano.5b03299
- Zhao Y, Dai X, Wang F, et al. Nanofabrication based on DNA nanotechnology. Nano Today. 2019;26:123–148. doi: 10.1016/j.nantod.2019.03.004
- Zhang J, Li Y, Zhang X, et al. Colloidal self-assembly meets nanofabrication: from two-dimensional colloidal crystals to nanostructure arrays. Adv Mater. 2010;22:4249–4269. doi: 10.1002/adma.201000755
- Service RF. How far can we push chemical self-assembly? Science. 2005;309:95–95. doi: 10.1126/science.309.5731.95
- Mathews KR, Mani E. Stabilizing ordered structures with single patch inverse patchy colloids in two dimensions. J Phys. 2021;33:195101. doi: 10.1088/1361-648X/abf0c0
- Dempster JM, Olvera De La Cruz M. Aggregation of heterogeneously charged colloids. ACS Nano. 2016;10:5909–5915. doi: 10.1021/acsnano.6b01218
- Fan X, Walther A. 1D colloidal chains: recent progress from formation to emergent properties and applications. Chem Soc Rev. 2022;51:4023–4074. doi: 10.1039/D2CS00112H
- Dijkstra M, Luijten E. From predictive modelling to machine learning and reverse engineering of colloidal self-assembly. Nature Mater. 2021;20:762–773. doi: 10.1038/s41563-021-01014-2
- Zhang J, Luijten E, Grzybowski BA, et al. Active colloids with collective mobility status and research opportunities. Chem Soc Rev. 2017;46:5551–5569. doi: 10.1039/C7CS00461C
- Marchetti MC, Joanny JF, Ramaswamy S, et al. Hydrodynamics of soft active matter. Rev Mod Phys. 2013;85:1143–1189. doi: 10.1103/RevModPhys.85.1143
- Chew PY, Reinhardt A. Phase diagrams—why they matter and how to predict them. J Chem Phys. 2023;158:030902. doi: 10.1063/5.0131028
- Rosenthal G, Gubbins KE, Klapp SHL. Self-assembly of model amphiphilic Janus particles. J Chem Phys. 2012;136:174901. doi: 10.1063/1.4707954
- Giacometti A, Lado F, Largo J, et al. Effects of patch size and number within a simple model of patchy colloids. J Chem Phys. 2010;132:174110. doi: 10.1063/1.3415490
- Craven NC, Gilmer JB, Spindel CJ, et al. Examining the self-assembly of patchy alkane-grafted silica nanoparticles using molecular simulation. J Chem Phys. 2021;154:034903. doi: 10.1063/5.0032658
- Kobayashi Y, Arai N. Self-assembly and viscosity behavior of Janus nanoparticles in nanotube flow. Langmuir. 2017;33:736–743. doi: 10.1021/acs.langmuir.6b02694
- Hu FF, Sun YW, Zhu YL, et al. Enthalpy-driven self-assembly of amphiphilic Janus dendrimers into onion-like vesicles: a Janus particle model. Nanoscale. 2019;11:17350–17356. doi: 10.1039/C9NR05885K
- Kern N, Frenkel D. Fluid–fluid coexistence in colloidal systems with short-ranged strongly directional attraction. J Chem Phys. 2003;118:9882–9889. doi: 10.1063/1.1569473
- Miller WL, Cacciuto A. Hierarchical self-assembly of asymmetric amphiphatic spherical colloidal particles. Phys Rev E. 2009;80:021404. doi: 10.1103/PhysRevE.80.021404
- Hong L, Cacciuto A, Luijten E, et al. Clusters of amphiphilic colloidal spheres. Langmuir. 2008;24:621–625. doi: 10.1021/la7030818
- Baran L, Borówko M, Rżysko W. Self-assembly of amphiphilic Janus particles confined between two solid surfaces. J Phys Chem C. 2020;124:17556–17565. doi: 10.1021/acs.jpcc.0c03214
- Preisler Z, Vissers T, Munaò G, et al. Equilibrium phases of one-patch colloids with short-range attractions. Soft Matter. 2014;10:5121–5128. doi: 10.1039/C4SM00505H
- Munaò G, Preisler Z, Vissers T, et al. Cluster formation in one-patch colloids: low coverage results. Soft Matter. 2013;9:2652. doi: 10.1039/c2sm27490f
- Vissers T, Preisler Z, Smallenburg F, et al. Predicting crystals of Janus colloids. J Chem Phys. 2013;138:164505. doi: 10.1063/1.4801438
- Sciortino F, Giacometti A, Pastore G. Phase diagram of Janus particles. Phys Rev Lett. 2009;103:237801. doi: 10.1103/PhysRevLett.103.237801
- Sciortino F, Giacometti A, Pastore G. A numerical study of one-patch colloidal particles: from square-well to Janus. Phys Chem Chem Phys. 2010;12:11869. doi: 10.1039/c0cp00504e
- Giacometti A, Gögelein C, Lado F, et al. From square-well to Janus: improved algorithm for integral equation theory and comparison with thermodynamic perturbation theory within the kern-frenkel model. J Chem Phys. 2014;140:094104. doi: 10.1063/1.4866899
- Reinhart WF, Panagiotopoulos AZ. Equilibrium crystal phases of triblock Janus colloids. J Chem Phys. 2016;145:094505. doi: 10.1063/1.4961869
- Bianchi E, Tartaglia P, Zaccarelli E, et al. Theoretical and numerical study of the phase diagram of patchy colloids: ordered and disordered patch arrangements. J Chem Phys. 2008;128:144504. doi: 10.1063/1.2888997
- Bianchi E, Largo J, Tartaglia P, et al. Phase diagram of patchy colloids: towards empty liquids. Phys Rev Lett. 2006;97:168301. doi: 10.1103/PhysRevLett.97.168301
- Preisler Z, Vissers T, Smallenburg F, et al. Crystals of Janus colloids at various interaction ranges. J Chem Phys. 2016;145:064513. doi: 10.1063/1.4960423
- Sato M. Effect of patch area and interaction length on clusters and structures formed by one-patch particles in thin systems. ACS Omega. 2020;5:28812–28822. doi: 10.1021/acsomega.0c04159
- Huang Z, Chen P, Yang Y, et al. Shearing Janus nanoparticles confined in two-dimensional space: reshaped cluster configurations and defined assembling kinetics. J Phys Chem Lett. 2016;7:1966–1971. doi: 10.1021/acs.jpclett.6b00724
- DeLacruz-Araujo RA, Beltran-Villegas DJ, Larson RG, et al. Rich Janus colloid phase behavior under steady shear. Soft Matter. 2016;12:4071–4081. doi: 10.1039/C6SM00183A
- Oh JS, Lee S, Glotzer SC, et al. Colloidal fibers and rings by cooperative assembly. Nat Commun. 2019;10:3936. doi: 10.1038/s41467-019-11915-1
- Nikoubashman A. Self-assembly of colloidal micelles in microfluidic channels. Soft Matter. 2017;13:222–229. doi: 10.1039/C6SM00766J
- Baran L, Borówko M, Rżysko W, et al. Amphiphilic Janus particles confined in symmetrical and Janus-like slits. ACS Omega. 2023;8:18863–18873. doi: 10.1021/acsomega.3c01180
- Bianchi E, Panagiotopoulos AZ, Nikoubashman A. Self-assembly of Janus particles under shear. Soft Matter. 2015;11:3767–3771. doi: 10.1039/C5SM00281H
- Sabapathy M, Ann Mathews KR, Mani E. Self-assembly of inverse patchy colloids with tunable patch coverage. Phys Chem Chem Phys. 2017;19:13122–13132. doi: 10.1039/C7CP00680B
- Sobrino Fernández M, Misko VR, Peeters FM. Self-assembly of Janus particles confined in a channel. Phys Rev E. 2014;89:022306. doi: 10.1103/PhysRevE.89.022306
- Li ZW, Lu ZY, Sun ZY, et al. Model, self-assembly structures, and phase diagram of soft Janus particles. Soft Matter. 2012;8:6693. doi: 10.1039/c2sm25397f
- Moghani MM, Khomami B. Self-assembly of spherical Janus particles in electrolytes. Soft Matter. 2013;9:4815. doi: 10.1039/c3sm27345h
- Safaei S, Todd C, Yarndley J, et al. Asymmetric assembly of lennard-jones Janus dimers. Phys Rev E. 2021;104:024602. doi: 10.1103/PhysRevE.104.024602
- Kharazmi A, Priezjev NV. Diffusion of a Janus nanoparticle in an explicit solvent: a molecular dynamics simulation study. J Chem Phys. 2015;142:234503. doi: 10.1063/1.4922689
- Kobayashi Y, Arai N, Nikoubashman A. Structure and shear response of Janus colloid–polymer mixtures in solution. Langmuir. 2020;36:14214–14223. doi: 10.1021/acs.langmuir.0c02308
- Hong L, Cacciuto A, Luijten E, et al. Clusters of charged Janus spheres. Nano Lett. 2006;6:2510–2514. doi: 10.1021/nl061857i
- De Araújo JLB, Munarin FF, Farias GA, et al. Structure and reentrant percolation in an inverse patchy colloidal system. Phys Rev E. 2017;95:062606. doi: 10.1103/PhysRevE.95.062606
- Bianchi E, Kahl G, Likos CN. Inverse patchy colloids: from microscopic description to mesoscopic coarse-graining. Soft Matter. 2011;7:8313. doi: 10.1039/c1sm05597f
- Cerbelaud M, Lebdioua K, Tran CT, et al. Brownian dynamics simulations of one-patch inverse patchy particles. Phys Chem Chem Phys. 2019;21:23447–23458. doi: 10.1039/C9CP04247D
- Zhang Z, Glotzer SC. Self-assembly of patchy particles. Nano Lett. 2004;4:1407–1413. doi: 10.1021/nl0493500
- Xu J, Wang Y, He X. Self-assembly of Janus ellipsoids: a Brownian dynamics simulation with a quantitative nonspherical-particle model. Soft Matter. 2015;11:7433–7439. doi: 10.1039/C5SM01667C
- Li YC, Zhang NB, Wei Z, et al. A computer simulation study of the hierarchical assembly behaviour of triblock patchy particles. Mol Simulat. 2019;45:759–767. doi: 10.1080/08927022.2019.1593976
- Safaei S, Archereau AYM, Hendy SC, et al. Molecular dynamics simulations of Janus nanoparticles in a fluid flow. Soft Matter. 2019;15:6742–6752. doi: 10.1039/C9SM00694J
- Safaei S, Hendy SC, Willmott GR. Stability of amphiphilic Janus dimers in shear flow: a molecular dynamics study. Soft Matter. 2020;16:7116–7125. doi: 10.1039/D0SM00871K
- Banik M, Sett S, Bakli C, et al. Substrate wettability guided oriented self assembly of Janus particles. Sci Rep. 2021;11:1182. doi: 10.1038/s41598-020-80760-w
- Koplik J, Maldarelli C. Molecular dynamics study of the translation and rotation of amphiphilic Janus nanoparticles at a vapor-liquid surface. Physical Review Fluids. 2019;4:044201. doi: 10.1103/PhysRevFluids.4.044201
- Chen Q, Bae SC, Granick S. Staged self-assembly of colloidal metastructures. J Am Chem Soc. 2012;134:11080–11083. doi: 10.1021/ja303434d
- Hagy MC, Hernandez R. Dynamical simulation of dipolar Janus colloids: equilibrium structure and thermodynamics. J Chem Phys. 2012;137:044505. doi: 10.1063/1.4737432
- Hagy MC, Hernandez R. Dynamical simulation of dipolar Janus colloids: dynamical properties. J Chem Phys. 2013;138:184903. doi: 10.1063/1.4803864
- Popov A, Hernandez R. Bottom-up construction of the interaction between Janus particles. J Phys Chem B. 2023;127:1664–1673. doi: 10.1021/acs.jpcb.2c07858
- Kobayashi Y, Arai N. Self-assembly of Janus nanoparticles with a hydrophobic hemisphere in nanotubes. Soft Matter. 2016;12:378–385. doi: 10.1039/C5SM01895A
- Hieronimus R, Raschke S, Heuer A. How to model the interaction of charged Janus particles. J Chem Phys. 2016;145:064303. doi: 10.1063/1.4960424
- Baowan D, Hill JM. Mathematical modeling of interaction energies between nanoscale objects: a review of nanotechnology applications. Adv Mech Eng. 2016;8:168781401667702. doi: 10.1177/1687814016677022
- Baowan D, Cox BJ, Hilder TA, et al. Modelling and mechanics of carbon-based nanostructured materials. Oxford, UK: William Andrew; 2017.
- Mathews Kalapurakal RA, Mani E. Orientation-dependent electrostatic interaction between inverse patchy colloids. Mol Simulat. 2022;48:176–184. doi: 10.1080/08927022.2021.1998487
- Labbé-Laurent M, Dietrich S. Critical Casimir interactions between Janus particles. Soft Matter. 2016;12:6621–6648. doi: 10.1039/C6SM00990E
- Squarcini A, Maciołek A, Eisenriegler E, et al. Critical Casimir interaction between colloidal Janus-type particles in two spatial dimensions. J Stat Mech. 2020;2020:043208. doi: 10.1088/1742-5468/ab7658
- Farahmand Bafi N, Nowakowski P, Dietrich S. Effective pair interaction of patchy particles in critical fluids. J Chem Phys. 2020;152:114902. doi: 10.1063/5.0001293
- Hagan MF, Elrad OM, Jack RL. Mechanisms of kinetic trapping in self-assembly and phase transformation. J Chem Phys. 2011;135:104115. doi: 10.1063/1.3635775
- Grzybowski BA, Fitzner K, Paczesny J, et al. From dynamic self-assembly to networked chemical systems. Chem Soc Rev. 2017;46:5647–5678. doi: 10.1039/C7CS00089H
- Iwashita Y, Kimura Y. Orientational order of one-patch colloidal particles in two dimensions. Soft Matter. 2014;10:7170–7181. doi: 10.1039/C4SM00932K
- Wagner N, Mewis J. Theory and applications of colloidal suspension rheology. Cambridge, UK: Cambridge University Press; 2021.
- Sciortino F. Entropy in self-assembly. La Rivista Del Nuovo Cimento. 2019;42:511–548.
- Mao X, Chen Q, Granick S. Entropy favours open colloidal lattices. Nature Mater. 2013;12:217–222. doi: 10.1038/nmat3496
- Chen Q, Whitmer JK, Jiang S, et al. Supracolloidal reaction kinetics of Janus spheres. Science. 2011;331:199–202. doi: 10.1126/science.1197451
- Smallenburg F, Sciortino F. Liquids more stable than crystals in particles with limited valence and flexible bonds. Nat Phys. 2013;9:554–558. doi: 10.1038/nphys2693
- Shin H, Schweizer KS. Theory of two-dimensional self-assembly of Janus colloids: crystallization and orientational ordering. Soft Matter. 2014;10:262–274. doi: 10.1039/C3SM52094C
- Kohl R, Corona E, Cheruvu V, et al. Fast and accurate solvers for simulating Janus particle suspensions in Stokes flow. Adv Comput Math. 2023;49:45. doi: 10.1007/s10444-023-10046-y
- Baran L, Borówko M, Rżysko W, et al. Self-assembly of Janus disks confined in a slit. J Chem Phys. 2019;151:104703. doi: 10.1063/1.5117887
- Kobayashi Y, Arai N, Nikoubashman A. Structure and dynamics of amphiphilic Janus spheres and spherocylinders under shear. Soft Matter. 2020;16:476–486. doi: 10.1039/C9SM01937E
- Mountain RD, Hatch HW, Shen VK. Molecular dynamics simulation of trimer self-assembly under shear. Fluid Phase Equilibria. 2017;440:87–94. doi: 10.1016/j.fluid.2017.02.017
- Molotilin TY, Lobaskin V, Vinogradova OI. Electrophoresis of Janus particles: a molecular dynamics simulation study. J Chem Phys. 2016;145:244704. doi: 10.1063/1.4972522
- Daghighi Y, Gao Y, Li D. 3D numerical study of induced-charge electrokinetic motion of heterogeneous particle in a microchannel. Electrochimica Acta. 2011;56:4254–4262. doi: 10.1016/j.electacta.2011.01.083
- Dhiman M, Gupta R, Reddy KA. Hydrodynamic interactions between two side-by-side Janus spheres. European J Mech - B/Fluids. 2021;87:61–74. doi: 10.1016/j.euromechflu.2021.01.006
- Fu SP, Ryham R, Quaife B, et al. Effects of tunable hydrophobicity on the collective hydrodynamics of Janus particles under flows. Physical Review Fluids. 2023;8:050501. doi: 10.1103/PhysRevFluids.8.050501
- Zhang Y, He X, Zhuo R, et al. Multivalent, multiflavored droplets by design. Proc Nat Acad Sci. 2018;115:9086–9091. doi: 10.1073/pnas.1718511115
- Oh JS, Yi GR, Pine DJ. Reconfigurable transitions between one- and two-dimensional structures with bifunctional DNA-Coated Janus colloids. ACS Nano. 2020;14:15786–15792. doi: 10.1021/acsnano.0c06846
- Goodwin JWJW. Colloids and interfaces with surfactants and polymers. 2nd ed. Chichester, UK: Wiley; 2009.
- Tsyrenova A, Miller K, Yan J, et al. Surfactant-mediated assembly of amphiphilic Janus spheres. Langmuir. 2019;35:6106–6111. doi: 10.1021/acs.langmuir.9b00500
- Sacanna S, Irvine WTM, Chaikin PM, et al. Lock and key colloids. Nature. 2010;464:575–578. doi: 10.1038/nature08906
- Feng L, Dreyfus R, Sha R, et al. DNA patchy particles. Adv Mater. 2013;25:2779–2783. doi: 10.1002/adma.201204864
- Xing H, Wang Z, Xu Z, et al. DNA-directed assembly of asymmetric nanoclusters using Janus nanoparticles. ACS Nano. 2012;6:802–809. doi: 10.1021/nn2042797
- Hayes OG, McMillan JR, Lee B, et al. DNA-encoded protein Janus nanoparticles. J Am Chem Soc. 2018;140:9269–9274. doi: 10.1021/jacs.8b05640
- Gröschel AH, Walther A, Löbling TI, et al. Guided hierarchical co-assembly of soft patchy nanoparticles. Nature. 2013;503:247–251. doi: 10.1038/nature12610
- Kang C, Honciuc A. Self-assembly of Janus nanoparticles into transformable suprastructures. J Phys Chem Lett. 2018;9:1415–1421. doi: 10.1021/acs.jpclett.8b00206
- Kang C, Honciuc A. Versatile triblock Janus nanoparticles: synthesis and self-assembly. Chem Mater. 2019;31:1688–1695. doi: 10.1021/acs.chemmater.8b05073
- Asai M, Cacciuto A, Kumar SK. Quantitative analogy between polymer-grafted nanoparticles and patchy particles. Soft Matter. 2015;11:793–797. doi: 10.1039/C4SM02295E
- Miller K, Tsyrenova A, Anthony SM, et al. Drying mediated orientation and assembly structure of amphiphilic Janus particles. Soft Matter. 2018;14:6793–6798. doi: 10.1039/C8SM01147H
- Iwashita Y, Kimura Y. Stable cluster phase of Janus particles in two dimensions. Soft Matter. 2013;9:10694. doi: 10.1039/c3sm52146j
- Yan J, Bloom M, Bae SC, et al. Linking synchronization to self-assembly using magnetic Janus colloids. Nature. 2012;491:578–581. doi: 10.1038/nature11619
- Takei H, Shimizu N. Gradient sensitive microscopic probes prepared by gold evaporation and chemisorption on latex spheres. Langmuir. 1997;13:1865–1868. doi: 10.1021/la9621067
- Bradley LC, Chen WH, Stebe KJ, et al. Janus and patchy colloids at fluid interfaces. Curr Opin Colloid Interface Sci. 2017;30:25–33. doi: 10.1016/j.cocis.2017.05.001
- Ge X, Geng Y, Chen J, et al. Smart amphiphilic Janus microparticles: one-step synthesis and self-assembly. Chemphyschem. 2018;19:2009–2013. doi: 10.1002/cphc.201700838
- Cui J, Long D, Shapturenka P, et al. Janus particle-based microprobes: determination of object orientation. Colloids Surf A Physicochem Eng Asp. 2017;513:452–462. doi: 10.1016/j.colsurfa.2016.11.017
- Wittmeier A, Leeth Holterhoff A, Johnson J, et al. Rotational analysis of spherical, optically anisotropic Janus particles by dynamic microscopy. Langmuir. 2015;31:10402–10410. doi: 10.1021/acs.langmuir.5b02864
- Anthony SM, Yu Y. Tracking single particle rotation: probing dynamics in four dimensions. Anal Methods. 2015;7:7020–7028. doi: 10.1039/C5AY00522A
- Kretzschmar I, Song JHK. Surface-anisotropic spherical colloids in geometric and field confinement. Curr Opin Colloid Interface Sci. 2011;16:84–95. doi: 10.1016/j.cocis.2011.01.002
- Chavez BL, Sosnowski KC, Bauer MJ, et al. Toward nanoscale multiferroic devices: magnetic field-directed self-assembly and chaining in Janus nanofibers. AIP Adv. 2018;8:056808. doi: 10.1063/1.5007706
- Swan JW, Bauer JL, Liu Y, et al. Directed colloidal self-assembly in toggled magnetic fields. Soft Matter. 2014;10:1102–1109. doi: 10.1039/C3SM52663A
- Martchenko I, Crassous JJ, Mihut AM, et al. Anisotropic magnetic particles in a magnetic field. Soft Matter. 2016;12:8755–8767. doi: 10.1039/C6SM01411A
- Butter K, Bomans P, Frederik P, et al. Direct observation of dipolar chains in iron ferrofluids by cryogenic electron microscopy. Nature Mater. 2003;2:88–91. doi: 10.1038/nmat811
- Azari A, Crassous JJ, Mihut AM, et al. Directed self-assembly of polarizable ellipsoids in an external electric field. Langmuir. 2017;33:13834–13840. doi: 10.1021/acs.langmuir.7b02040
- Crassous JJ, Mihut AM, Wernersson E, et al. Field-induced assembly of colloidal ellipsoids into well-defined microtubules. Nat Commun. 2014;5:5516. doi: 10.1038/ncomms6516
- Knapp EM, Dagastine RR, Tu RS, et al. Effect of orientation and wetting properties on the behavior of Janus particles at the air–water interface. ACS Appl Mater Inter. 2020;12:5128–5135. doi: 10.1021/acsami.9b21067
- Geiger P, Dellago C. Neural networks for local structure detection in polymorphic systems. J Chem Phys. 2013;139:164105. doi: 10.1063/1.4825111
- Long AW, Ferguson AL. Rational design of patchy colloids via landscape engineering. Mol Syst Des Eng. 2018;3:49–65. doi: 10.1039/C7ME00077D
- Ma Y, Ferguson AL. Inverse design of self-assembling colloidal crystals with omnidirectional photonic bandgaps. Soft Matter. 2019;15:8808–8826. doi: 10.1039/C9SM01500K