ABSTRACT
Asexual spores, called conidia, are key reproductive fungal particles that enable survival in harsh environmental conditions or host systems. The conidia can infect humans, animals, and plants to cause various fungal diseases. Transcription factors, including VosA, WetA, and SscA, have key roles in conidia formation and long-term survival in Aspergillus nidulans. Herein, we report the pleiotropic functions of SscA in the conidia of the human pathogen A. fumigatus. The deletion of sscA increased conidia formation despite decreased fungal growth. Absence of sscA impaired long-term survival and reduced spore resistance to various stresses, including heat, UV, and oxidation. Transcriptomic analyses showed that SscA involved the mRNA expression of cell wall organisation-related genes. Importantly, the sscA deletion mutant conidia contained an increased amount of β-glucan and chitin compared to wild type conidia. In addition, conidial gliotoxin production was decreased in the sscA deletion strain. Overall, SscA has pleiotropic roles in conidia formation, maturation and dormancy and mycotoxin production in A. fumigatus.
1. Introduction
The Aspergillus species are saprotrophic filamentous fungi that decay organic materials for absorption of nutrients. These species are globally ubiquitous and are primarily spread by airborne asexual spores (conidia). Aspergillus fumigatus is the main opportunistic human pathogen among Aspergillus species. Most healthy people are unaffected by airborne A. fumigatus conidia, but people with impaired immunity can be threatened by A. fumigatus conidia, which are potent infectious particles in humans. The conidia (2–3 μm in diameter) can be inhaled into the lung thereby bypassing the immune defence mechanism; germination of conidia forms fungal hyphae to extend their niche and produce various secondary metabolites. Depending on the health of the immune system, A. fumigatus can cause symptoms ranging from mild fever and cough to severe and life-threatening diseases, including invasive pulmonary aspergillosis (IPA), aspergilloma, chronic pulmonary aspergillosis (CPA), allergic bronchopulmonary aspergillosis, and severe asthma with fungal sensitisation (Latge Citation1999; Cramer et al. Citation2011; van de Veerdonk et al. Citation2017; Earle et al. Citation2023). Therefore, it is necessary to understand A. fumigatus conidia to enable control of conidium-based A. fumigatus infections.
The bluish-green-coloured conidia of A. fumigatus start to generate from the stalks, followed by vesicles, metulae, and phialides. Asymmetric mitotic divisions of phialides arise in young conidia on conidiophores, which mature by coordinating the conidial cell wall compositions and cytoplasmic organelles (Ghiorse and Edwards Citation1973; Yu Citation2010). Separated from the conidiophores, conidia remain viable in their resting state for at least one year at room temperature until meeting favourable conditions (Lamarre et al. Citation2008). Dormant conidia store various transcripts required for rapid translation activity to enable spore germination (Lamarre et al. Citation2008; Wang et al. Citation2021). Conidia can also survive under hostile conditions, such as thermal, oxidative, and radiative stresses (Baltussen et al. Citation2019; Son et al. Citation2023b). Under these conditions, various regulators are involved in conidial survival. For example, velvet regulators VosA and VelB are essential for responding to oxidative and radiative stresses (Park et al. Citation2012). The mitogen-activated protein kinase SakA is required for tolerating osmotic and oxidative stress, cell wall-disturbing stress and heat shock (Bruder Nascimento et al. Citation2016). The bZIP transcription factor (TF) AtfA plays important roles in conidial viability under oxidative and thermal stress, dehydration and low temperatures (Hagiwara et al. Citation2014). The Myb-type TF MybA maintains long-term viability and controls trehalose and cell wall biosynthetic genes in conidia (Valsecchi et al. Citation2017). Knowledge of these genetic regulators, including TFs, kinases and phosphatases, in conidiogenesis, remains insufficient despite recent studies in the area, and further study is required to understand how fungi use conidia to proliferate and survive (Park and Yu Citation2016; Son et al. Citation2023b).
The cell wall of intact conidia is mainly composed of β-1,3-glucan, chitin, α-1,3-glucan, galactomannan and melanin, and the surface is covered with a rodlet layer (Valiante et al. Citation2015; Kumari et al. Citation2021). The most abundant polysaccharides of the cell wall are β-1,3-glucan and chitin. The β-1,3-glucan is branched and linked to chitin and galactomannan to make the conidia rigid (Fontaine et al. Citation2000). Several regulators are required to correctly organise β-1,3-glucan or chitin biosynthesis in the cell wall. β-1,3-glucan synthase (FksA) and β-1,3-glucanosyltransferases (Gel family) are necessary for glucan biosynthesis, and a total of nine chitin synthases are needed for chitin biogenesis in A. fumigatus (Fontaine et al. Citation2000; Brauer et al. Citation2023; Liu et al. Citation2023). The cell wall integrity signalling pathway (Bck1, Mkk, and MpkA) is involved in maintaining normal cell walls, and the downstream TFs RlmA and HsfA regulate cell wall integrity and remodelling by controlling glucan and chitin metabolism (Valiante et al. Citation2015; Rocha et al. Citation2020; Fabri et al. Citation2021). The conidial wall is a protective physical blockade that enables conidia to tolerate and adapt to external stressors, such as desiccation, oxidative stress, high temperatures and even hypoxic conditions in pulmonary tissue (Garcia-Rubio et al. Citation2019). Therefore, it is necessary to study regulators that coordinate conidial wall integrity.
Our previous studies found a gene sscA (spore-specific C2H2 A) which is commonly highly expressed in conidia of three Aspergillus species (A. nidulans, A. flavus, and A. fumigatus). The deletion of sscA resulted in defective conidia formation, impaired spore viability and increased spore stress sensitivity in A. nidulans. SscA also contributes to cell wall integrity and metabolism activity in A. nidulans conidia (Son et al. Citation2023a). In addition, homology of SscA in A. fumigatus exerted conserved roles in A. nidulans. However, SscA in conidia has not been studied in the human pathogenic fungus A. fumigatus. Herein, we characterised the roles of SscA (Afu3g09820) in conidia formation, conidial wall integrity, and gliotoxin production in A. fumigatus.
2. Materials and methods
2.1. Strains, media, and culture conditions
All A. fumigatus strains used in this study are shown in . A. fumigatus AF293 was used as the control strain, and A. fumigatus AF293.1 was used as the parental strain to construct deletion mutants. A. fumigatus strains were grown on minimal media with 0.1% yeast extract (MMY) as described previously (Park et al. Citation2012). To assay the number of conidia in wild-type (WT, AF293), sscA-deleted (ΔsscA) (TYE53.1) and complemented (Cʹ sscA) (TYE61.1) strains, approximately 106 spores, were point-inoculated onto solid MMY and incubated at 37 °C for 4 days. For plasmid construction, Escherichia coli DH5α was grown in Luria-Bertani medium (BD, Difco, USA) with 100 μg/mL of ampicillin (Thermo Scientific, USA).
Table 1. Aspergillus fumigatus strains used in this study.
2.2. Construction of deletion mutants and complemented strains
The oligonucleotides used in this study are listed in . For generating the sscA-deletion mutant, the double-joint PCR method was explored (Yu et al. Citation2004). The fragments containing regions flanking the 5ʹ and 3ʹ ends of the sscA gene were amplified from A. fumigatus AF293 genomic DNA (gDNA) with OHS1724, OHS1725, OHS1726, and OHS1727 primers. The A. nidulans pyrG marker was amplified from A. nidulans FGSC4 gDNA with OHS2008 and OHS2009. All fragments were linked and amplified using OHS1728 and OHS1729 primers. The resulting PCR cassette was introduced into the recipient pyrG− strain (A. fumigatus AF293.1) using a PEG-mediated transformation method. At least three independent deletion strains were constructed and verified by PCR and restriction enzymes (Figure S1).
Table 2. Oligonucleotides used in this study.
For generating sscA-complemented strains, the open reading frame region of the sscA gene was amplified from A. fumigatus AF293 gDNA with primer set OHS1826 and OHS1827, digested with PstI and cloned into pYES1 containing the pyrithiamine-resistant gene (ptrA) as a selectable marker. The cloned plasmid pYE12.1 was transformed into the recipient sscA strain (TYE53.1). The sscA-complemented transformants were selected in MMY supplemented with pyrithiamine (1 μg/mL) and confirmed by PCR and reverse-transcription-quantitative PCR (RT-qPCR) (Figure S1).
2.3. Phylogenetic analyses
The protein sequence of A. fumigatus SscA was retrieved from the FungiDB platform, and homologous proteins were identified with Basic Local Alignment Search Tool (BLASTP) searches of the National Center for Biotechnology Information (NCBI) database. A total of amino acid sequences of 80 Aspergillus species, including A. fumigatus, were aligned using Clustal W, and a phylogenetic tree was constructed with MEGA X software. The tree was generated based on the neighbour-joining method with 1,000 bootstrap replicates.
2.4. Transcriptome analysis
For the transcriptomic study of A. fumigatus WT and ΔsscA conidia, each strain was streaked onto solid MMY and grown at 37 °C for 2 days. Conidia of each strain were then suspended with 0.02% Triton X-100 (Thermo Scientific, USA) and filtered with Miracloth (Merck, USA).
Total RNA extraction was performed as previously described (Son and Park Citation2020). All samples were isolated using a Mini-bead beater (BioSpec Products Inc., USA) and TRIzol (GeneAll Biotechnology, Republic of Korea), and the extracted RNA samples were dissolved in diethylpyrocarbonate (DEPC)-treated water (Bioneer, Republic of Korea). Total RNA was treated with RQ1 RNase-free DNase (Promega, USA) and purified using the RNeasy Mini Kit (Qiagen, Germany). RNA sequencing was performed by Theragen Bio (Suwon, Republic of Korea). Briefly, three biological replicates of each strain were sequenced on Illumina Novaseq 6000, and reads were annotated with the A. fumigatus AF293 transcriptome using the aligner STAR v.2.3.0e software. The DESeq2 method was used for evaluating and normalising differentially expressed genes (DEGs). DEGs were screened with | log2(fold change)|≥1 and p value < 0.05, and gene ontology (GO) analyses were performed using the R package and FungiDB database.
2.5. RT-qPCR analysis
RT-qPCR was performed as previously described (Son and Park Citation2020). The extracted RNA samples were used for the synthesis of cDNA with GoScript reverse transcriptase (Promega, USA). RT-qPCR was performed with iTaq Universal SYBR Green Supermix (BioRad, USA), synthesised cDNA and gene-specific primers on a CFX96 Touch Real-Time PCR (BioRad, USA). The expression levels of target genes were normalised to the expression levels of β-actin, an endogenous control, and calculated using the 2−ΔΔCT method. Results are representative of three independent experimental replicates, and the primers used for quantitative PCR are listed in .
2.6. Spore viability and stress tolerance
For evaluating the long-term spore viability, 2-, 15-, and 30-day-old conidia of each strain were collected and counted using a haemocytometer. Approximately 100 conidia were spread onto solid MMY and incubated at 37 °C for 2 days. The relative survival rate was calculated as the ratio of the number of viable colonies in the 2-, 15-, and 30-day-grown conidia to the number of viable colonies in the 2-day-grown conidia.
To test spore thermal or oxidative stress tolerance, 2-day-old conidia of each strain were collected and counted using a haemocytometer. Conidia diluted by 103 were heated at 55 °C for 30 or 60 min or incubated with 0.05 mol/L H2O2. Approximately 100 conidia of each strain were spread onto solid MMY and incubated at 37 °C for 2 days (Park et al. Citation2012).
To test spore UV stress tolerance, 2-day-old conidia of each strain were collected and counted using a haemocytometer. Conidia diluted by 102 of each strain were spread onto solid MMY. Plates were irradiated directly with 50 J/m2 of UV light (254 nm) using a UV crosslinker (Spectrolinker XL-1000 UV crosslinker, Spectronics Corporation, USA) incubated at 37 °C for 2 days. The relative survival rate was calculated as the ratio of the number of viable colonies in the treated sample to the number of viable colonies in the untreated control. Spore viability and stress tolerance assays were replicated at least three times.
2.7. Spore β-glucan and chitin analysis
β-glucan in spores was assayed using a Glucatell ® assay kit (Associates of Cape Cod Inc., USA) as described previously (Park et al. Citation2015). The 2-day-old conidia of each strain were collected, diluted by 103, and resuspended in 25 μL. Each sample was mixed with Glucatell ® reagent and incubated at 37 °C for 30 min. The reaction was stopped using diazo coupling reagents, and the optical density of each sample was measured at 540 nm. The spore β-glucan assay was replicated at least three times.
Chitin was extracted from conidia and analysed as previously described (Lehmann and White Citation1975) with some modifications. The 2-day-old (2 × 109) conidia of each strain were collected, resuspended with 3% sodium dodecyl sulphate (Bioneer, Republic of Korea) and heated at 100 °C for 15 min. After being washed with ddH2O, saturated potassium hydroxide (Thermo Scientific, USA) was added and heated at 100 °C for 1 h. Then, 75% ice-cold ethanol (Merck) and 5% Celite 545 suspension (Samchun, Republic of Korea) were added and centrifuged at 4 °C. Each sample was washed with 40% ice-cold ethanol and ddH2O and adjusted to pH 2. After centrifuging at 4 °C, the supernatant was decanted and the pellet was suspended in the same volume of water: 5% sodium nitrite (Sigma, USA): 5% potassium hydrogen sulphate (Junsei Chemical, Japan). Samples were incubated at room temperature for 15 min and centrifuged at 4 °C, and 20 μL of 12.5% ammonium sulfamate (Sigma, USA) was added, and the mixture was incubated at room temperature for 5 min. After that, 20 μL of 0.5% 3-methylbenzthiazolinone-2-hydrazone (Thermo Scientific, USA) were added and incubated at 100 °C for 3 min. Finally, 20 μL of 0.83% ferric chloride (Sigma, USA) was added and incubated at room temperature for 30 min, and the optical density of each sample was measured at 650 nm. The negative control was distilled water, and the positive control was 1% glucosamine (Thermo Scientific, USA). The chitin assay was replicated at least three times.
2.8. Spot assay for cell wall stresses
For a spot dilution assay, 2-day-old conidia of each strain were collected and counted using a haemocytometer. Conidia suspensions of each strain, starting with 2 × 106 conidia/mL, were serially ten-fold diluted and spotted on solid MMY containing cell wall-stressing reagents: 10, 50, or 100 μg/mL of Congo red (CR) and calcofluor white (CFW). All plates were incubated at 37 °C for 48 h and then photographed (Schruefer et al. Citation2021).
2.9. Gliotoxin (GT) analysis
GT production was analysed by thin-layer chromatography (TLC) as described previously with minor modifications (Xiao et al. Citation2010). The 7-day-grown conidia (1 × 1010) of each strain were collected and homogenised using a Mini-Bead beater (BioSpec Products Inc.) with 0.3 mL of glass beads (Daihan Scientific, Republic of Korea) and 1 mL of chloroform (Sigma, USA). The organic phase was mixed with ddH2O and centrifuged. The purified organic phase was transferred to a new glass vial and dried at 65 °C overnight. Samples were resuspended with 0.1 mL of methanol (Honeywell, USA), spotted onto a TLC silica plate coated with the fluorescent indicator F254 (Merck Millipore, USA), and resolved in TLC plates containing toluene (Daejung, Republic of Korea): ethyl acetate (Daejung, Republic of Korea): acetic acid (Thermo Fisher, USA) (5:4:1, v/v/v). The TLC plate was exposed and captured under UV light at 254 nm. The relative intensities of GT were calculated using the ImageJ software.
2.10. In vivo A. fumigatus infection
Six-week-old Balb/c female mice were used for the experiment after a one-week stabilisation period (n = 7). For immunosuppression, 250 mpk of cyclophosphamide was orally administered before infection (3 days, 1 day before), and 125 mpk of cyclophosphamide was orally administered after infection (1, 6, and 12 days after). One day before infection, 250 mpk of cortisone acetate was also orally administered. 1 × 107 conidia of each strain were anaesthetized with isoflurane and then nasally inhaled. After infection, the condition of the mice was observed every day, and the survival rate of the mice was expressed as percent survival. All experiments followed the experimental ethics guidelines by the Institutional Animal Care and Use Committee of the Experimental Animal Center at Jeonbuk National University (Approval number JBNU 2023-132). The survival curves were analysed using the Log-rank (Mantel-Cox).
2.11. Statistical analysis
Statistical differences between WT and ΔsscA strains were evaluated with Student’s unpaired t-test. Data are reported as mean ± standard deviation. p values < 0.05 were significant.
3. Results
3.1. SscA in Aspergillus species
The open reading frame of sscA (Afu3g09820), comprising 2,100 nucleotides with two exons and an intron, was predicted to encode a protein with 679 amino acids and an estimated mass of 73.2 kDa. SscA has been indicated to be highly conserved in diverse phylum of fungi (Son et al. Citation2023a). To understand the role of SscA in Aspergillus species, we identified the homologues of SscA among 80 Aspergillus species via the BLASTP tool in NCBI and analysed the phylogenetic relationship (Table S1). SscA homologues were conserved in most Aspergillus species and subdivided along the subgenus and section (). All 80 homologues of SscA were predicted to contain a Cys2His2 zinc finger domain with the C-X(5)-C-X(12)-H-X(3)-H present in the N-terminus in all proteins, although some amino acids differed according to section (Figure S2).
Figure 1. In silico analysis of SscA in Aspergillus species. a phylogenetic tree of SscA homologues identified in Aspergillus species. Protein sequences were obtained from the NCBI database, and alignment was performed with clustal W. The tree was generated via MEGA X to examine SscA homologues. The tree based on the neighbour-joining method was replicated 1,000 times, and the scale represented the number of substitutions per site.
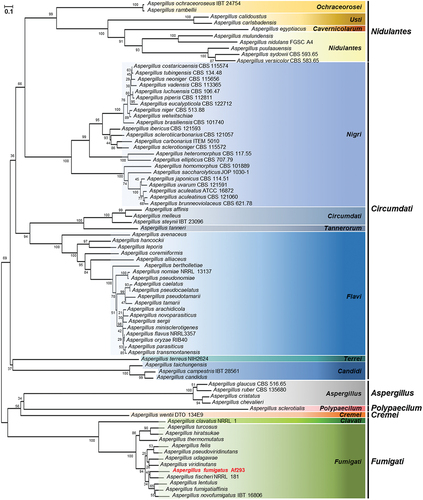
3.2. SscA affects vegetative growth and conidia formation
To investigate the functions of SscA in A. fumigatus, ΔsscA and Cʹ sscA strains were point-inoculated onto solid MMY and incubated at 37 °C for 4 days. As shown in , the deletion of sscA resulted in the formation of light-coloured colony. While the fungal colony of the ΔsscA mutant was slightly decreased in size compared with that of the WT and Cʹ sscA strains, the number of conidia per cm2 in ΔsscA strain was increased compared with that in the WT and Cʹ sscA strains (). However, the germination rate of ΔsscA conidia was not different compared with other strains (Figure S3). These results suggested that SscA is required for normal fungal growth and conidiation in A. fumigatus.
Figure 2. Functions of SscA in Aspergillus fumigatus development. (a) Photographs of colonies of WT, ΔsscA and Cʹ sscA strains point-inoculated onto solid MMY and grown at 37 °C for 4 days. (b) Quantitative analysis of fungal growth of strains shown in (a); error bars indicate the standard error of the mean in three biological replicates (**p < 0.01). (c) Quantitative analysis of asexual spore formation of the strains shown in (a); error bars indicate the standard error of the mean in three biological replicates (**p < 0.01).
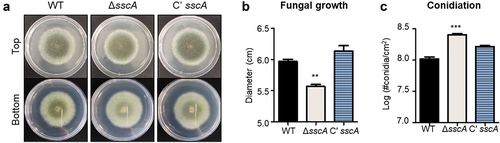
3.3. SscA is involved in long-term viability and stress tolerance
Our previous study demonstrated that SscA is required for conidial dormancy and stress resistance in the model organism A. nidulans (Son et al. Citation2023a). To further explore whether the functions of SscA are conserved in the human pathogen A. fumigatus conidia, we assayed the viability with 2-, 15-, and 30-old conidia of each strain. Although the survival rates of the WT and Cʹ sscA strains did not fluctuate at 15 and 30 days, the survival rates of ΔsscA mutant showed a significant decrease over time (). Next, we examined the conidial survival rates under various stresses. The survival rate of ΔsscA conidia was similar to that of WT and Cʹ sscA conidia when heated at 55 °C for 30 min (). However, the viability of ΔsscA conidia was significantly reduced compared with that of the WT and Cʹ sscA strains when heated for 60 min (). In addition, ΔsscA conidia were more sensitive than either WT or Cʹ sscA conidia when treated with oxidative and UV stresses (). Thus, SscA plays an important role in spore viability and stress tolerance in A. fumigatus conidia.
Figure 3. Roles of SscA in Aspergillus fumigatus conidia. (a) The relative survival rates of WT, ΔsscA and Cʹ sscA conidia grown for 2-, 15-, or 30-days (**p < 0.01, *p < 0.05). (b–d) The relative conidial survival rates of the designated strains treated with thermal stress (55 °C) for 30 or 60 min (b), oxidative stress (0.05 mol/L H2O2) for 30 min (c), or UV stress (50 J/m2) (d); error bars indicate the standard error of the mean in three biological replicates (**p < 0.01, *p < 0.05).
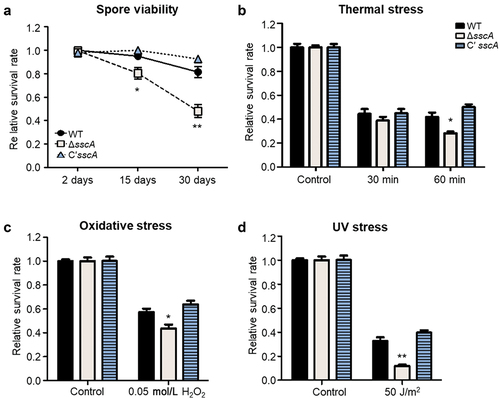
3.4. Genome-wide transcriptome analysis of SscA in A. fumigatus conidia
To gain further insight into the functions of SscA in A. fumigatus conidia, we conducted RNA sequencing with WT and ΔsscA conidia grown for 2 days. Among the predicted 10,130 A. fumigatus genes, a total of 2,156 (|fold change| ≥ 2.0; p value < 0.05) were differentially expressed between WT and ΔsscA conidia (). The expression of approximately 12.7% and 8.5% of the genes were up- and down-regulated in ΔsscA conidia, respectively. We then performed GO term enrichment analyses based on the FungiDB platform (, Table S2). The up-regulated genes were associated with the developmental process, anatomical structure development, secondary metabolite biosynthetic process, cell wall organisation or biogenesis, external encapsulating structure organisation, hyphal growth, and conidium development, whereas the down-regulated genes were involved in the alpha amino acid metabolic process, organic cyclic compound catabolic process, nucleoside metabolic process, protein localisation to membrane, and the endoplasmic reticulum. These suggested that SscA influences mRNA expression of various genes associated with development, cellular metabolism, and cell wall composition in A. fumigatus conidia.
3.5. SscA is required for beta-glucan and chitin biosynthesis
SscA is indispensable for normal cell wall structure in A. nidulans conidia (Son et al. Citation2023a), and our transcriptomic data indicated that deletion of sscA affected gene expression of cell wall biogenesis in A. fumigatus conidia, suggesting that SscA can affect β-glucan and chitin biosynthesis. First, we further checked RNA-seq results and found that the mRNA expression levels of β-glucan biosynthetic genes in WT and ΔsscA conidia (Baltussen et al. Citation2019; Garcia-Rubio et al. Citation2019) were more highly expressed in ΔsscA conidia than in those of the WT conidia (). We then verified the RNA-seq results through the RT-qPCR analysis and confirmed that the transcripts of fksA and gelA, the key β-glucan biosynthetic genes, were upregulated in the ΔsscA conidia (). Furthermore, the β-glucan level in ΔsscA conidia was significantly increased compared with those of the WT and Cʹ sscA conidia ().
Figure 5. Effect of SscA on key conidial wall components in Aspergillus fumigatus. (a) Heatmap showing the DEGs among β-glucan biosynthetic genes in WT and ΔsscA conidia. (b) The relative mRNA expression levels of major β-glucan biosynthetic genes (fksA and gelA) in WT, ΔsscA, and Cʹ sscA conidia as assessed by RT-qPCR (*p < 0.05). (c) Amount of β-glucan in conidia of WT, ΔsscA, and Cʹ sscA strains (**p < 0.01). (d) Heatmap showing the DEGs among chitin biosynthetic genes in WT and ΔsscA conidia. (e) The relative mRNA expression levels of four chitin biosynthetic genes (chsA, chsB, chsC, and chsE) in WT, ΔsscA, and Cʹ sscA conidia as assessed by RT-PCR (*p < 0.05). (f) Amount of chitin in conidia of WT, ΔsscA, and Cʹ sscA strains (***p < 0.001). (g) Sensitivity of the designated strains to cell wall disturbing agents. The error bars indicate the standard error of the mean in three biological replicates.
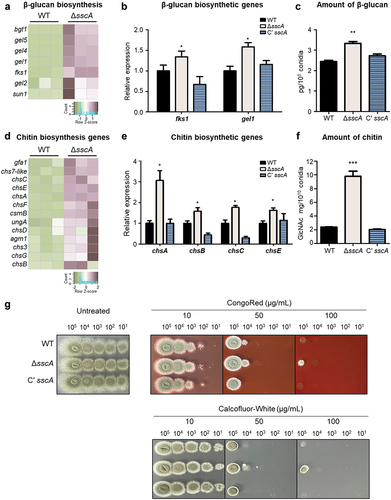
We then analysed the mRNA levels of chitin biosynthetic genes and found that the expression of most of the chitin biosynthetic genes was upregulated in the ΔsscA conidia () . The RT-qPCR analysis verified that the relative expression levels of four chs genes were significantly increased in the ΔsscA conidia compared with that in the WT and Cʹ sscA conidia (). We also determined that the amount of hydrolysed N-acetylglucosamine, a monomer unit of chitin, in the ΔsscA conidia was approximately 4-fold that in other strains (), suggesting that SscA is required for proper chitin biosynthesis in conidia.
We then investigated whether SscA influences sensitivity to well-known cell wall-stressing agents such as CR and CFW that mainly bind to β-1,3-glucan and chitin, respectively (Ram and Klis Citation2006). Sensitivity to CR and CFW was determined by a spot assay. The serially diluted, 2-day-grown conidia were spotted onto MMY medium with CR or CFW and incubated at 37 °C for 2 days. The sensitivity of the ΔsscA strains was similar to that of WT and Cʹ sscA strains at a low concentration of CR (10 μg/mL) or CFW (10 μg/mL) (). However, the ΔsscA strain showed a slightly elevated resistance to 50 μg/mL of CR and CFW, and only the ΔsscA strain developed colonies under high concentrations of CR (100 μg/mL) or CFW (100 μg/mL). The mRNA expression levels of α-glucan biosynthetic genes, another fundamental component of the fungal cell wall, were also upregulated in ΔsscA conidia (Figure S4). Overall, these results suggest that SscA is critical for conidial wall composition by coordinating expression of cell wall organisation-related genes in A. fumigatus.
3.6. SscA contributes to GT production
GT is a sulphur-containing secondary metabolite that is the major and most potent mycotoxin produced by A. fumigatus (de Castro et al. Citation2022). The biosynthesis and secretion of GT are regulated by the GT biosynthetic gene cluster including the final oxidoreductase GliT, efflux pump GliA, and TF RglT (Schrettl et al. Citation2010; Wang et al. Citation2014; Ries et al. Citation2020). RNA-seq data found that deletion of sscA affected mRNA expression of genes involved in secondary metabolism ( and Table S3). To investigate the functions of SscA in GT production, we collected 2- or 7-day old conidia (1010) of each strain and extracted the fungal metabolites. The total organic metabolites were separated from fungal metabolites in a thin-layer silica gel. The ΔsscA conidia produced slightly less GT than either WT or Cʹ sscA conidia (). The relative band intensity of GT in ΔsscA was significantly decreased compared with that of WT or Cʹ sscA strains. Furthermore, we confirmed that mRNA expression of GT-related genes (gliA, gliT, and rglT) was downregulated in ΔsscA compared with that in WT and Cʹ sscA (). The 7-day-old conidia showed similar results, with the ΔsscA strain exhibiting decreased production of GT and mRNA expression of GT synthetic-related genes (). To further examine the function of SscA in GT biosynthesis in mycelia, WT and mutant strains were cultured in liquid MMY media and checked GT production. The ΔsscA exhibited a considerably reduced abundance of GT at 7 days compared with that of the other strains (Figure S5). Overall, these suggested that SscA affects GT production in A. fumigatus.
Figure 6. Functions of SscA in gliotoxin (GT) production. (a) TLC of gliotoxin from the 2-day-grown conidia of WT, ΔsscA, and Cʹ sscA strains. The right bar plot indicates the relative band intensity of gliotoxin shown in TLC plate; error bars indicate the standard error of the mean in three biological replicates (**p < 0.01). (b) The relative mRNA expression levels of gliotoxin biosynthetic genes (gliA, gliT, and rglT) in WT, ΔsscA and Cʹ sscA conidia grown for 2 days (***p < 0.001, **p < 0.01). (c) TLC of gliotoxin from the 7-day-grown conidia of WT, ΔsscA, and Cʹ sscA strains. The right bar plot indicates the relative band intensity of gliotoxin shown in TLC plate; error bars indicate the standard error of the mean in three biological replicates (**p < 0.01). (d) The relative mRNA expression levels of gliotoxin biosynthetic genes (gliA, gliT, and rglT) in WT, ΔsscA, and Cʹ sscA conidia grown for 7 days (**p < 0.01, *p < 0.05).
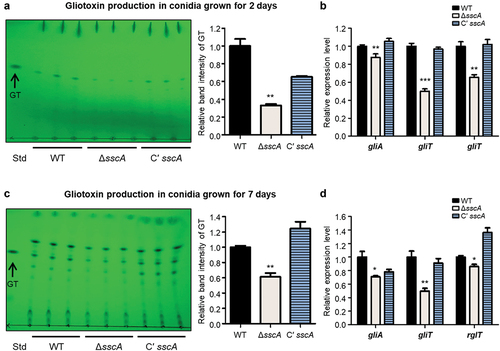
3.7. SscA influences the pathogenicity of A. fumigatus
A. fumigatus is the primary human pathogenic fungus that causes symptoms ranging from cough and fever to severe aspergillosis, depending on the immune status of the host (Dagenais and Keller Citation2009). In other to investigate the functions of SscA on the virulence of A. fumigatus, we collected 2-day old conidia (107) of each strain and intranasally infected them to mice immunosuppressed by cyclophosphamide and cyclophosphamide (). As a result, the mortality of mice infected with ΔsscA conidia was rather higher than that of mice infected with WT strain, but this was not statistically significant (). Therefore, this result proposes that SscA does not seem to have a significant effect on fungal pathogenicity in hosts.
Figure 7. Functions of SscA in the virulence of Aspergillus fumigatus. (a) Schematic representation of fungal infection in the mice model. (b) Survival rate of A. fumigatus WT, ΔsscA, and Cʹ sscA strains in mice model. Statistical differences were calculated using the Log-rank (mantel-cox) test (WT vs. KO, 0.1328; WT vs. complemented, 0.6767; KO vs. complemented, 0.0483).
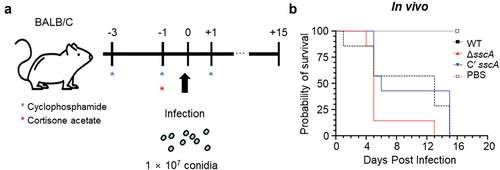
4. Discussion
A. fumigatus is a primary causative agent of fungal-related diseases. Host species are continually exposed as A. fumigatus is inhaled through the respiratory tract and can infect the lungs, causing various diseases and even death (Lin et al. Citation2001; Brown et al. Citation2012). Conidia are the main infectious particles that are easily dispersed through the air, and therefore it is worthwhile seeking to control conidia viability and resistance (Nayak et al. Citation2018). We previously demonstrated that the spore-specific C2H2 zinc finger SscA TF is essential for conidial formation, maturation and dormancy in A. nidulans (Son et al. Citation2023a). In the current study, we investigated the roles of SscA in A. fumigatus conidia. The absence of sscA induced abnormal conidia formation, reduced long-term spore viability, and increased conidial stress sensitivity in A. fumigatus. Transcriptome studies indicated that SscA plays a key role in maintaining proper conidial wall components by regulating the expression of cell wall biosynthetic genes. In addition, SscA affected GT production in A. fumigatus conidia.
Previous studies have revealed that the defective phenotypes of ΔAnisscA in asexual development and conidial physiology are restored when AfuSscA is expressed in A. nidulans (Son et al. Citation2023a). Herein, we investigated whether such multiple roles of SscA in conidiogenesis are indeed conserved in A. fumigatus. First, as in A. nidulans, ΔsscA conidia showed defective long-term viability compared with WT and Cʹ sscA conidia in A. fumigatus. Deletion of sscA increased the sensitivity of conidia to thermal, oxidative and UV stresses (). Although the A. nidulans ΔsscA strain did not affect fungal growth and showed defective conidia formation, the A. fumigatus ΔsscA strain exhibited reduced fungal growth and increased conidia formation (). Collectively, the functions of SscA on conidial viability and stress response are conserved but have different roles in fungal growth and conidia formation in different Aspergillus species.
There are many virulence factors, including conidial tolerance, conidial surface and cell wall, pigment, and secondary metabolites in A. fumigatus (Hohl and Feldmesser Citation2007; Raffa et al. Citation2019). Our RNA-sequencing analysis showed that a variety of genes associated with the secondary metabolite biosynthetic process was upregulated in ΔsscA conidia (). To predict the effect of SscA on secondary metabolism, we further investigated all DEGs in 33 secondary metabolite gene clusters of A. fumigatus (Table S3). There were five upregulated secondary metabolites where the ratio of DEGs per secondary metabolite gene cluster was >60% (Figure S6): cluster 2 (nidulanin-like), cluster 3 (ferricrocin), cluster 15, cluster 18 (trypacidin), and cluster 26 (fumiquinazoline C). Although the bioactivities of the other metabolites have not been investigated yet, trypacidin is known as a conidia-bound metabolite that lowers the recognition or uptake of conidia by macrophages (Mattern et al. Citation2015). Fumiquinazoline, which accumulated in A. fumigatus conidia, is also a cytotoxic peptidyl alkaloid with antibacterial and antifungal activities. Taken together, we speculated that ΔsscA conidia may cause hypervirulence in humans, animals, and microorganisms by regulating gene expression of genes involved in specific secondary metabolites.
A. fumigatus is a saprophytic fungus that adapts and survives under various stressful conditions, including in different environments and hosts. To sustain their viability, A. fumigatus uses several strategies that involve trehalose, heat-shock proteins, and cell wall integrity (Al-Bader et al. Citation2010; Tiwari et al. Citation2015; Brown and Goldman Citation2016; Baltussen et al. Citation2019). To understand the regulatory roles of SscA in stress tolerance, we examined the amount of trehalose in the conidia of each strain. The amount of trehalose was slightly increased in ΔsscA conidia compared with that in conidia of WT and Cʹ sscA strains (Data not shown). To gain insights into the stress sensitivity of ΔsscA conidia, we also analysed the expression levels of heat-shock proteins (HSPs) based on transcriptome data. Among the various sizes of HSPs, the expression of relatively small HSPs (sHSPs) was downregulated in ΔsscA conidia (Figure S7A). Several studies have indicated that sHSPs play major roles in various stress responses in fungi (Tiwari et al. Citation2015; Wu et al. Citation2016; Dev Citation2021). Furthermore, the expression of DNA repair-related genes was downregulated in ΔsscA conidia (Figure S7B). Altogether, SscA controls resistance to various stresses by regulating the expression of stress-related genes in A. fumigatus conidia.
Fungal cell walls with both flexible and rigid properties can be remodelled depending on the internal and external environment and play as protective barriers that withstand extremely unfavourable conditions (Latge Citation2007; Gow et al. Citation2017). However, it is difficult to demonstrate a direct relationship between the cell wall organisation and sensitivity to various stresses. Deletion of sscA resulted in increased β-glucans and chitin contents and increased resistance in cell wall perturbing agents (). However, ΔsscA showed increased sensitivity in thermal, oxidative, and radiative stresses (). Similar to SscA, deletion of nsdC exhibited increased cell wall thickness and mannose and galactose, but increased sensitivity to cell wall stressors (Alves de Castro et al. Citation2021). The ΔrlmA also showed increased cell wall thickness, and β-1,3-glucan and chitin levels, but it was more sensitive to cell wall damaging compounds (CR, CFW, and caspofungin), cell membrane disturbing agent (SDS), chelating agent (EDTA), and oxidative stressors (paraquat, menadione, and t-butyl hydroperoxide) (Rocha et al. Citation2016). The ΔnikA exerted a thinner cell wall and reduced galactosamine contents compared to WT and C’ nikA, but deletion of nikA strain was more resistance to CR, CFW and micafungin and less resistance to high osmotic stresses including NaCl and KCl (Hagiwara et al. Citation2013). Overall, these imply that the alteration of conidial wall components influences the different responses under varying stresses, suggesting adaptation to the extracellular environment in A. fumigatus involves not only cell wall composition but also other robust and complex signalling pathways.
Importantly, SscA is a highly conserved TF in Aspergillus species () as well as in other fungi (Son et al. Citation2023a), and its homologous proteins influence fungal pathogenicity. Usv101 in Cryptococcus neoformans is a key regulator for full pathogenesis that regulates melanin production and capsule growth. A usv101Δ strain exhibited increased capsule thickness and size, thereby preventing phagocytotic engulfing and delaying mouse infection and death (Gish et al. Citation2016). In Candida albicans, Bcr1 is required for the fungal burden by regulating biofilm formation in mice models (Dwivedi et al. Citation2011; Fanning et al. Citation2012). Nsf1 in Fusarium graminearum and Gcf6 in Magnaporthe oryzae play vital roles in full virulence in wheat and rice, respectively (Cao et al. Citation2016; Shi et al. Citation2021). Similar to other fungal species, SscA has an effect on the pathogenicity of A. fumigatus in mice models (). Previous studies demonstrated that ΔdvrA (ΔsscA) had increased virulence in the nonneutropenic mice model as well as the Galleria melonella model and suggested that the hypervirulence in mice infected with ΔdvrA was induced by augmented pulmonary fungal burdens (Ejzykowicz et al. Citation2010). However, DvrA did not affect pathogenicity in the neutropenic mice model, which differed from our results. We speculate on two possibilities for these distinct results. First, we intranasally injected fungal spores directly, whereas the Ejzykowicz group infected mice in fungal spores-aerosolized chambers for 1 h; thus, because of these different experimental methods, the actual concentration of infected fungal spores may have varied. Second, differences were present in the amount and frequency of immunosuppressive drugs used. Nevertheless, with the previous and current studies, we suggest that AfuSscA, unlike other SscA homologues in other fungi, is important for the regulation of pathogenicity in hosts.
Notably, A. fumigatus produces multiple virulence factors, including an external encapsulating structure and fungal mycotoxins and exhibits thermotolerance, antioxidant defence mechanisms and adaptation to hypoxic conditions (Tomee and Kauffman Citation2000; Hohl and Feldmesser Citation2007; Abad et al. Citation2010). The fungal cell wall is closely related to the external environment and can evoke the host immune system when recognised as pathogen-associated molecular patterns. For example, β-glucan is recognised by the C-type lectin receptor dectin-1 and induces an inflammatory response (Hohl et al. Citation2005; Hopke et al. Citation2018). Chitin stimulates inflammatory immune reactions through recognition via the toll-like receptor 2 and dectin-1 (van de Veerdonk et al. Citation2017; Elieh Ali Komi et al. Citation2018). The infected conidia can be engulfed by phagocytes (mainly alveolar macrophages), which subsequently induce inflammatory reactions and excessive immune responses ranging from asthma to CPA and IPA. Herein, we determined that SscA is critical for regulating correct conidial wall dynamics in A. fumigatus. As shown in , the amounts of β-glucan and chitin, the main cell wall constitutions, were significantly increased in the conidia of ΔsscA strain. Previous studies suggested that large-sized cells of C. neoformans and C. albicans persist in the host by avoiding phagocytosis (Zaragoza et al. Citation2010; Min et al. Citation2020). We suggest that the rigid ΔsscA conidia with accumulated glucan and chitin may impede engulfment by phagocytes and could subsequently evade the immune response to germinate into hyphae and form a biofilm, thereby leading to hypervirulence in the mice model (van de Veerdonk et al. Citation2017). However, further studies are required to fully comprehend the roles of SscA in the interaction between A. fumigatus spores and host immune cells.
Overall, our phenotypic and transcriptomic studies revealed that the spore-specific TF SscA has pleiotropic roles in the regulation of conidia formation, spore stress response and longevity, conidial wall organisation and mycotoxin production in A. fumigatus. The importance of SscA in conidial properties is clear but has yet to be fully investigated. Therefore, further studies are needed to identify direct targets of SscA and elucidate the detailed molecular mechanisms of SscA in A. fumigatus conidia.
Supplemental Material
Download MS Word (1.8 MB)Acknowledgments
The work by SYE was supported by the National Research Foundation of Korea (NRF) grant to SYE funded by the Korean government (MSIP: 2021R1A6A3A13044577). The work by HSP was supported by the National Research Foundation of Korea (NRF) grant to HSP funded by the Korean government (MSIP: 2020R1C1C1004473). This research was supported by Biological Materials Specialized Graduate Program through the Korea Environmental Industry & Technology Institute (KEITI) funded by the Ministry of Environment (MOE) and supported by the Korea Basic Science Institute (National Research Facilities and Equipment Center) grant funded by the Ministry of Education (2021R1A6C101A416).
Disclosure statement
No potential conflict of interest was reported by the author(s).
Data availability statement
All RNA-seq data files are available at the NCBI Sequence Read Archive (SRA) database under the accession number PRJNA983063 for WT and ΔsscA of Aspergillus fumigatus conidia RNA-seq.
Supplementary Material
Supplemental data for this article can be accessed online at https://doi.org/10.1080/21501203.2023.2294061
Additional information
Funding
References
- Abad A, Fernandez-Molina JV, Bikandi J, Ramirez A, Margareto J, Sendino J, Hernando FL, Ponton J, Garaizar J, Rementeria A. 2010. What makes Aspergillus fumigatus a successful pathogen? Genes and molecules involved in invasive aspergillosis. Rev Iberoam Micol. 27(4):155–182. doi: 10.1016/j.riam.2010.10.003.
- Al-Bader N, Vanier G, Liu H, Gravelat FN, Urb M, Hoareau CM, Campoli P, Chabot J, Filler SG, Sheppard DC. 2010. Role of trehalose biosynthesis in Aspergillus fumigatus development, stress response, and virulence. Infect Immun. 78(7):3007–3018. doi: 10.1128/IAI.00813-09.
- Alves de Castro P, Valero C, Chiaratto J, Colabardini AC, Pardeshi L, Pereira Silva L, Almeida F, Campos Rocha M, Nascimento Silva R, Malavazi I, et al. 2021. Novel biological functions of the NsdC transcription factor in Aspergillus fumigatus. mBio. 12(1):e03102–20. doi:10.1128/mBio.03102-20.
- Baltussen TJH, Zoll J, Verweij PE, Melchers WJG. 2019. Molecular mechanisms of conidial germination in Aspergillus spp. Microbiol Mol Biol Rev. 84(1):e00049–19. doi: 10.1128/MMBR.00049-19.
- Brauer VS, Pessoni AM, Freitas MS, Cavalcanti-Neto MP, Ries LNA, Almeida F. 2023. Chitin biosynthesis in Aspergillus species. J Fungi (Basel). 9(1):89. doi: 10.3390/jof9010089.
- Brookman JL, Denning DW. 2000. Molecular genetics in Aspergillus fumigatus. Curr Opin Microbiol. 3(5):468–474. doi: 10.1016/S1369-5274(00)00124-7.
- Brown GD, Denning DW, Gow NA, Levitz SM, Netea MG, White TC. 2012. Hidden killers: human fungal infections. Sci Transl Med. 4(165):165rv113. doi: 10.1126/scitranslmed.3004404.
- Brown NA, Goldman GH. 2016. The contribution of Aspergillus fumigatus stress responses to virulence and antifungal resistance. J Microbiol. 54(3):243–253. doi: 10.1007/s12275-016-5510-4.
- Bruder Nascimento AC, Dos Reis TF, de Castro, Hori JI, Bom VL, de Assis LJ, Ramalho LN, Rocha MC, Malavazi I, Brown NA, et al. 2016. Mitogen activated protein kinases SakA HOG1 and MpkC collaborate for Aspergillus fumigatus virulence. Mol Microbiol. 100(5):841–859. doi:10.1111/mmi.13354.
- Cao H, Huang P, Zhang L, Shi Y, Sun D, Yan Y, Liu X, Dong B, Chen G, Snyder JH, et al. 2016. Characterization of 47 Cys2 -His2 zinc finger proteins required for the development and pathogenicity of the rice blast fungus Magnaporthe oryzae. New Phytol. 211(3):1035–1051. doi:10.1111/nph.13948.
- Cramer RA, Rivera A, Hohl TM. 2011. Immune responses against Aspergillus fumigatus: what have we learned? Curr Opin Infect Dis. 24(4):315–322. doi: 10.1097/QCO.0b013e328348b159.
- Dagenais TR, Keller NP. 2009. Pathogenesis of Aspergillus fumigatus in invasive Aspergillosis. Clin Microbiol Rev. 22(3):447–465. doi: 10.1128/CMR.00055-08.
- de Castro PA, Colabardini AC, Moraes M, Horta MAC, Knowles SL, Raja HA, Oberlies NH, Koyama Y, Ogawa M, Gomi K, et al. 2022. Regulation of gliotoxin biosynthesis and protection in Aspergillus species. PLoS Genet. 18(1):e1009965. doi:10.1371/journal.pgen.1009965.
- Dev R. 2021. Exploring small heat shock proteins (sHsps) for targeting drug resistance in Candida albicans and other pathogenic fungi. J Pure Appl Microbio. 15(1):20–28. doi: 10.22207/JPAM.15.1.42.
- Dwivedi P, Thompson A, Xie Z, Kashleva H, Ganguly S, Mitchell AP, Dongari-Bagtzoglou A, Davis D. 2011. Role of Bcr1-activated genes Hwp1 and Hyr1 in Candida albicans oral mucosal biofilms and neutrophil evasion. PLoS One. 6(1):e16218. doi: 10.1371/journal.pone.0016218.
- Earle K, Valero C, Conn DP, Vere G, Cook PC, Bromley MJ, Bowyer P, Gago S. 2023. Pathogenicity and virulence of Aspergillus fumigatus. Virulence. 14(1):2172264. doi: 10.1080/21505594.2023.2172264.
- Ejzykowicz DE, Solis NV, Gravelat FN, Chabot J, Li X, Sheppard DC, Filler SG. 2010. Role of Aspergillus fumigatus DvrA in host cell interactions and virulence. Eukaryot Cell. 9(10):1432–1440. doi: 10.1128/EC.00055-10.
- Elieh Ali Komi D, Sharma L, Dela Cruz CS. 2018. Chitin and its effects on inflammatory and immune responses. Clin Rev Allergy Immunol. 54(2):213–223. doi: 10.1007/s12016-017-8600-0.
- Fabri J, Rocha MC, Fernandes CM, Persinoti GF, Ries LNA, da Cunha AF, Goldman GH, Del Poeta M, Malavazi I, Cunha AFD. 2021. The heat shock transcription factor HsfA is essential for thermotolerance and regulates cell wall integrity in Aspergillus fumigatus. Front Microbiol. 12:656548. doi: 10.3389/fmicb.2021.656548.
- Fanning S, Xu W, Solis N, Woolford CA, Filler SG, Mitchell AP. 2012. Divergent targets of Candida albicans biofilm regulator Bcr1 in vitro and in vivo. Eukaryot Cell. 11(7):896–904. doi: 10.1128/EC.00103-12.
- Fontaine T, Simenel C, Dubreucq G, Adam O, Delepierre M, Lemoine J, Vorgias CE, Diaquin M, Latge JP. 2000. Molecular organization of the alkali-insoluble fraction of Aspergillus fumigatus cell wall. J Biol Chem. 275(36):27594–27607. doi: 10.1074/jbc.M909975199.
- Garcia-Rubio R, de Oliveira HC, Rivera J, Trevijano-Contador N, de Oliveira HC. 2019. The fungal cell wall: Candida, Cryptococcus, and Aspergillus species. Front Microbiol. 10:2993. doi: 10.3389/fmicb.2019.02993.
- Ghiorse WC, Edwards MR. 1973. Ultrastructure of Aspergillus fumigatus conidia development and maturation. Protoplasma. 76(1):49–59. doi: 10.1007/BF01279672.
- Gish SR, Maier EJ, Haynes BC, Santiago-Tirado FH, Srikanta DL, Ma CZ, Li LX, Williams M, Crouch EC, Khader SA, et al. 2016. Computational analysis reveals a key regulator of cryptococcal virulence and determinant of host response. mBio. 7(2):e00313–00316. doi:10.1128/mBio.00313-16.
- Gow NAR, Latge JP, Munro CA, Heitman J. 2017. The fungal cell wall: Structure, biosynthesis, and function. Microbiol Spectr. 5(3):FUNK-0035–2016. doi: 10.1128/microbiolspec.FUNK-0035-2016.
- Hagiwara D, Suzuki S, Kamei K, Gonoi T, Kawamoto S. 2014. The role of AtfA and HOG MAPK pathway in stress tolerance in conidia of Aspergillus fumigatus. Fungal Genet Biol. 73:138–149. doi: 10.1016/j.fgb.2014.10.011.
- Hagiwara D, Takahashi-Nakaguchi A, Toyotome T, Yoshimi A, Abe K, Kamei K, Gonoi T, Kawamoto S, Goldman GH. 2013. NikA/TcsC histidine kinase is involved in conidiation, hyphal morphology, and responses to osmotic stress and antifungal chemicals in Aspergillus fumigatus. PLoS One. 8(12):e80881. doi: 10.1371/journal.pone.0080881.
- Hohl TM, Feldmesser M. 2007. Aspergillus fumigatus: principles of pathogenesis and host defense. Eukaryot Cell. 6(11):1953–1963. doi: 10.1128/EC.00274-07.
- Hohl TM, Van Epps HL, Rivera A, Morgan LA, Chen PL, Feldmesser M, Pamer EG, Cormack B. 2005. Aspergillus fumigatus triggers inflammatory responses by Stage-specific β-glucan display. PLoS Pathog. 1(3):e30. doi: 10.1371/journal.ppat.0010030.
- Hopke A, Brown AJP, Hall RA, Wheeler RT. 2018. Dynamic fungal cell wall architecture in stress adaptation and immune evasion. Trends Microbiol. 26(4):284–295. doi: 10.1016/j.tim.2018.01.007.
- Kumari A, Tripathi AH, Gautam P, Gahtori R, Pande A, Singh Y, Madan T, Upadhyay SK. 2021. Adhesins in the virulence of opportunistic fungal pathogens of human. Mycology. 12(4):296–324. doi: 10.1080/21501203.2021.1934176.
- Lamarre C, Sokol S, Debeaupuis JP, Henry C, Lacroix C, Glaser P, Coppee JY, Francois JM, Latge JP. 2008. Transcriptomic analysis of the exit from dormancy of Aspergillus fumigatus conidia. Bmc Genom. 9(1):417. doi: 10.1186/1471-2164-9-417.
- Latge JP. 1999. Aspergillus fumigatus and aspergillosis. Clin Microbiol Rev. 12(2):310–350. doi: 10.1128/CMR.12.2.310.
- Latge JP. 2007. The cell wall: a carbohydrate armour for the fungal cell. Mol Microbiol. 66(2):279–290. doi: 10.1111/j.1365-2958.2007.05872.x.
- Lehmann PF, White LO. 1975. Chitin assay used to demonstrate renal localization and cortisone-enhanced growth of Aspergillus fumigatus mycelium in mice. Infect Immun. 12(5):987–992. doi: 10.1128/iai.12.5.987-992.1975.
- Lin SJ, Schranz J, Teutsch SM. 2001. Aspergillosis case-fatality rate: Systematic review of the literature. Clin Infect Dis. 32(3):358–366. doi: 10.1086/318483.
- Liu Z, Valsecchi I, Le Meur RA, Simenel C, Guijarro JI, Comte C, Muszkieta L, Mouyna I, Henrissat B, Aimanianda V, et al. 2023. Conidium specific polysaccharides in Aspergillus fumigatus. J Fungi (Basel). 9(2):155. doi:10.3390/jof9020155.
- Mattern DJ, Schoeler H, Weber J, Novohradska S, Kraibooj K, Dahse HM, Hillmann F, Valiante V, Figge MT, Brakhage AA. 2015. Identification of the antiphagocytic trypacidin gene cluster in the human-pathogenic fungus Aspergillus fumigatus. Appl Microbiol Biotechnol. 99(23):10151–10161. doi: 10.1007/s00253-015-6898-1.
- Min K, Neiman AM, Konopka JB. 2020. Fungal pathogens: shape-shifting invaders. Trends Microbiol. 28(11):922–933. doi: 10.1016/j.tim.2020.05.001.
- Nayak AP, Croston TL, Lemons AR, Goldsmith WT, Marshall NB, Kashon ML, Germolec DR, Beezhold DH, Green BJ. 2018. Aspergillus fumigatus viability drives allergic responses to inhaled conidia. Ann Allergy Asthma Immunol. 121(2):200–210 e202. doi: 10.1016/j.anai.2018.04.008.
- Park HS, Bayram O, Braus GH, Kim SC, Yu JH. 2012. Characterization of the velvet regulators in Aspergillus fumigatus. Mol Microbiol. 86(4):937–953. doi: 10.1111/mmi.12032.
- Park HS, Man Yu Y, Lee MK, Jae Maeng P, Chang Kim S, Yu JH. 2015. Velvet-mediated repression of beta-glucan synthesis in Aspergillus nidulans spores. Sci Rep. 5:10199. doi: 10.1038/srep10199.
- Park HS, Yu JH. 2016. Developmental regulators in Aspergillus fumigatus. J Microbiol. 54(3):223–231. doi: 10.1007/s12275-016-5619-5.
- Raffa N, Keller NP, Sheppard DC. 2019. A call to arms: mustering secondary metabolites for success and survival of an opportunistic pathogen. PLoS Pathog. 15(4):e1007606. doi: 10.1371/journal.ppat.1007606.
- Ram AF, Klis FM. 2006. Identification of fungal cell wall mutants using susceptibility assays based on calcofluor white and Congo red. Nat Protoc. 1(5):2253–2256. doi: 10.1038/nprot.2006.397.
- Ries LNA, Pardeshi L, Dong Z, Tan K, Steenwyk JL, Colabardini AC, Ferreira Filho JA, de Castro PA, Silva LP, Preite NW, et al. 2020. The Aspergillus fumigatus transcription factor RglT is important for gliotoxin biosynthesis and self-protection, and virulence. PLoS Pathog. 16(7):e1008645. doi:10.1371/journal.ppat.1008645.
- Rocha MC, Fabri JH, Franco de Godoy K, Alves de Castro P, Hori JI, Ferreira da Cunha A, Arentshorst M, Ram AF, van den Hondel CA, Goldman GH, et al. 2016. Aspergillus fumigatus MADS-Box transcription factor rlmA is required for regulation of the cell wall integrity and virulence. G3 (Bethesda). 6(9):2983–3002. doi:10.1534/g3.116.031112.
- Rocha MC, Fabri J, Simoes IT, Silva-Rocha R, Hagiwara D, da Cunha AF, Goldman GH, Canovas D, Malavazi I. 2020. The cell wall integrity pathway contributes to the early stages of Aspergillus fumigatus asexual development. Appl Environ Microbiol. 86(7):e02347–19. doi: 10.1128/AEM.02347-19.
- Schrettl M, Carberry S, Kavanagh K, Haas H, Jones GW, Oʹbrien J, Nolan A, Stephens J, Fenelon O, Doyle S, et al. 2010. Self-protection against gliotoxin — A component of the gliotoxin biosynthetic cluster, gliT, completelyprotects Aspergillus fumigatus against exogenous gliotoxin. PLoS Pathog. 6(6):e1000952. doi: 10.1371/journal.ppat.1000952.
- Schruefer S, Bohmer I, Dichtl K, Spadinger A, Kleinemeier C, Ebel F. 2021. The response regulator Skn7 of Aspergillus fumigatus is essential for the antifungal effect of fludioxonil. Sci Rep. 11(1):5317. doi: 10.1038/s41598-021-84740-6.
- Shi DY, Ren WC, Wang J, Zhang J, Mbadianya JI, Mao XW, Chen CJ. 2021. The transcription factor FgNsf1 regulates fungal development, virulence and stress responses in Fusarium graminearum. J Integr Agr. 20(8):2156–2169. English. doi:10.1016/S2095-3119(20)63339-1.
- Son YE, Park HS. 2020. Genome wide analysis reveals the role of vada in stress response, germination, and sterigmatocystin production in Aspergillus nidulans conidia. Microorganisms. 8(9):1319. doi: 10.3390/microorganisms8091319.
- Son YE, Yu JH, Park HS 2023a. The novel spore-specific regulator SscA controls Aspergillus conidiogenesis. mBio. e01840–01823.
- Son YE, Yu JH, Park HS. 2023b. Regulators of the asexual life cycle of Aspergillus nidulans. Cells. 12(11):1544. doi: 10.3390/cells12111544.
- Tiwari S, Thakur R, Shankar J. 2015. Role of heat-shock proteins in cellular function and in the biology of fungi. Biotechnol Res Int. 2015:132635. doi: 10.1155/2015/132635.
- Tomee JF, Kauffman HF. 2000. Putative virulence factors of Aspergillus fumigatus. Clin Exp Allergy. 30(4):476–484. doi: 10.1046/j.1365-2222.2000.00796.x.
- Valiante V, Macheleidt J, Foge M, Brakhage AA. 2015. The Aspergillus fumigatus cell wall integrity signaling pathway: Drug target, compensatory pathways, and virulence. Front Microbiol. 6:325. doi: 10.3389/fmicb.2015.00325.
- Valsecchi I, Sarikaya-Bayram O, Wong Sak Hoi J, Muszkieta L, Gibbons J, Prevost MC, Mallet A, Krijnse-Locker J, Ibrahim-Granet O, Mouyna I, et al. 2017. MybA, a transcription factor involved in conidiation and conidial viability of the human pathogen Aspergillus fumigatus. Mol Microbiol. 105(6):880–900. doi:10.1111/mmi.13744.
- van de Veerdonk FL, Gresnigt MS, Romani L, Netea MG, Latge JP. 2017. Aspergillus fumigatus morphology and dynamic host interactions. Nat Rev Microbiol. 15(11):661–674. doi: 10.1038/nrmicro.2017.90.
- Wang DN, Toyotome T, Muraosa Y, Watanabe A, Wuren T, Bunsupa S, Aoyagi K, Yamazaki M, Takino M, Kamei K. 2014. GliA in Aspergillus fumigatus is required for its tolerance to gliotoxin and affects the amount of extracellular and intracellular gliotoxin. Med Mycol. 52(5):506–518. doi: 10.1093/mmy/myu007.
- Wang F, Sethiya P, Hu X, Guo S, Chen Y, Li A, Tan K, Wong KH. 2021. Transcription in fungal conidia before dormancy produces phenotypically variable conidia that maximize survival in different environments. Nat Microbiol. 6(8):1066–1081. doi: 10.1038/s41564-021-00922-y.
- Wu J, Wang M, Zhou L, Yu D. 2016. Small heat shock proteins, phylogeny in filamentous fungi and expression analyses in Aspergillus nidulans. Gene. 575(2 Pt 3):675–679. doi: 10.1016/j.gene.2015.09.044.
- Xiao P, Shin KS, Wang T, Yu JH. 2010. Aspergillus fumigatus flbB encodes two basic leucine zipper domain (bZIP) proteins required for proper asexual development and gliotoxin production. Eukaryot Cell. 9(11):1711–1123. doi: 10.1128/EC.00198-10.
- Xue T, Nguyen CK, Romans A, Kontoyiannis DP, May GS. 2004. Isogenic auxotrophic mutant strains in the Aspergillus fumigatus genome reference strain AF293. Arch Microbiol. 182(5):346–353. doi: 10.1007/s00203-004-0707-z.
- Yu JH. 2010. Regulation of development in Aspergillus nidulans and Aspergillus fumigatus. Mycobiology. 38(4):229–237. doi: 10.4489/MYCO.2010.38.4.229.
- Yu JH, Hamari Z, Han KH, Seo JA, Reyes-Dominguez Y, Scazzocchio C. 2004. Double-joint PCR: A PCR-based molecular tool for gene manipulations in filamentous fungi. Fungal Genet Biol. 41(11):973–981. doi: 10.1016/j.fgb.2004.08.001.
- Zaragoza O, Garcia-Rodas R, Nosanchuk JD, Cuenca-Estrella M, Rodriguez-Tudela JL, Casadevall A, Mitchell AP. 2010. Fungal cell gigantism during mammalian infection. PLoS Pathog. 6(6):e1000945. doi: 10.1371/journal.ppat.1000945.