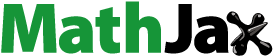
Abstract
The thermodynamics of mass transfer during gaseous carburization and its overall efficiency across various alloyed steels were investigated to discern the influence of alloy content. Quantitative analyses were conducted on the coefficients of mass transfer and carbon diffusivities for EN3, EN35, 20MnCr5, and EN353 steels at a carburization temperature of 930 °C. The study revealed distinct trends in mass transfer dynamics and carbon diffusion rates among the different alloyed steels. For instance, nickel majorly an austenite stabilizer, was found to enhance carbon diffusion in gamma iron, with observed coefficients of mass transfer ranging from 8.85 × 10−6 to 1.87 × 10−5 cm/s. However, EN35 steel exhibited slower mass transfer rates due to weaker atomic interactions, resulting in lower observed coefficients of mass transfer. Conversely, chromium and molybdenum, known carbide-forming elements, facilitated carbon transfer from the gaseous environment to the steel case in 20MnCr5 and EN353 steels, with observed coefficients of mass transfer ranging from 1.39 × 10−5 to 1.55 × 10−5 cm/s. Additionally, the study compared observed carbon diffusivities with those predicted by DICTRA (Diffusion Controlled TRAnsformation) kinetic and thermodynamic datasets, demonstrating excellent consistency. These findings underscore the significance of considering alloy content in achieving consistent carburizing outcomes and suggest potential modifications to existing carburizing methods to improve diffusion depth homogeneity. Overall, the study provides valuable insights into the impact of alloy elements on the carburization process and offers implications for optimizing carburizing practices in alloy steel manufacturing.
1. Introduction
There are various surface hardening treatments available for the production of aerospace and automotive components with hard and wear-resistant surfaces (cases) and tough cores, in which gas carburizing is widely used, cost effective, and desirable. Considering its global usage, this method faces various obstacles in terms of process control and diffusion depth (carbon) uncertainty (Boubaker et al., Citation2003; Ma et al., Citation2022). The carburizing capability of steel is affected by the design of the furnace, process parameters such as the alloying elements present, composition of the carrier gas, carburizing temperature, and holding duration. The impact of these process parameters on the performance of carburization has been the subject of many studies. Nevertheless, considerable fluctuations in the effective diffusion depth and surface carbon concentration cannot be fully explained, even using a well-controlled method (Jung et al., Citation2009; Ochsner et al., Citation2004; Wei et al., Citation2014; Abdulrazzaq Jabbar & Kadhim, Citation2020; Gu et al., Citation2014; Wu et al., Citation2011). As a result, the objective of this research is to better understand how the chemical composition of steel affects the kinetics of carbon diffusion into the surface, the gas carburizing process, and the overall behavior of steel during carburization. The goal of this study is to explore the impact of common alloying elements on the carbon diffusion rate in austenite and the coefficient mass transfer in a gas-carburizing environment.
Many studies have examined the effect of the alloy composition on the rate of gas carburization. Ernst et al. (Citation2011) developed a thermodynamic model that determines the influence of alloying elements present in low-carbon steels on carbon diffusion in austenite for various ternary Fe-C-X systems. The model demands a homogeneous chemical potential for carbon, which is not discussed for the two phases, and assumes a stationary ferrite and austenite interface, resulting in a metastable equilibrium situation known as restricted para-equilibrium. Other studies have also explored how the alloy composition affects the diffusivity and mobility of carbon in austenite. The study of diffusion pairs forms the foundation of the majority of these studies. Owing to the assumption of average surface density, there is some ambiguity when using such models for gas carburizing (Ernst et al., Citation2011; Jiang et al., Citation2019; Sharghi-Moshtaghin et al., Citation2010; Heintzberger, Citation2020; Kim et al., Citation2011; Trotea et al., Citation2018). As a result, the most popular method for accounting for the impact of steel composition includes ‘alloying’ the effective carbon potential in the gas environment. Egawa et al. (Citation2010) investigated the improvement in austenitic stainless steels through low-temperature plasma nitriding and carburizing processes, creating a hardened layer known as the ‘S-phase’. The thickness of these layers increased at higher process temperatures, with AISI316 steel having the thickest layer. However, above a critical temperature, the corrosion resistance decreases owing to the precipitation of chromium nitride or carbide. The critical temperature varied with the substrate material during nitriding but remained consistent during carburizing. While most S-phase layers had lower corrosion resistance than untreated stainless steels, AISI316 and JIS-SUS304J3 steels carburized at 400 °C exhibited excellent corrosion resistance. Furthermore, all stainless steels demonstrated significantly improved wear resistance following these treatments. Rowan and Sisson (Citation2009) studied the effect of alloy composition on the gas-carburizing performance of AISI 1018, 4820, 5120, and 8620 steels. It was found that steels with higher concentrations of austenite-stabilizing elements had increased carbon diffusivity in austenite but reduced carbon transfer kinetics, leading to lower weight gain. Conversely, steels with carbide-forming elements enhanced the mass-transfer coefficient and accelerated the carbon profile evolution despite their lower carbon diffusivities. The experimental results are in good agreement with the DICTRA database. In summary, considering concentration-dependent mass-transfer coefficients and carbon diffusivities in various alloy steels can help improve case depth uniformity in carburizing processes.
Despite producing satisfactory findings, this empirically determined correction factor does not clearly link the alloy composition to the coefficients of mass transfer from the carburizing environment to the steel surface or austenitic carbon diffusivity. Consequently, the calculations and data analysis in this study were based on a modified technique of direct flux integration to investigate the nature of the interactions. This approach, which has already been shown to be effective, allows for the computation of mass transfer coefficients and carbon diffusivity in austenite using a straightforward experimental setup.
Moreover, this study significantly contributes to quality control and consistency. The variations in the carbon concentration profiles and weight gains for different steel compositions during carburization, as elucidated in this work, provide practical insights for ensuring product quality. Industries can use this information to implement rigorous quality control measures, reduce the likelihood of defects, and minimize the need for costly reworks. This not only saves resources but also enhances the overall reliability and reputation of manufacturing processes. shows a schematic representation of the study.
The primary novelty of this study lies in its quantitative analysis of the interplay between alloy composition and gas carburization in various steel types. Quantifying the mass transfer coefficients and carbon diffusivities offers a clear understanding of how different alloy elements affect the carburization process. This study highlights the importance of considering the alloy composition when carburizing steel, as it influences the mass transfer and carbon diffusion rates. This quantitative approach provides valuable insights for achieving consistent and controlled carburization outcomes, emphasizing the significance of alloy compositions in industrial applications.
2. Gas carburizing thermodynamics and mass transfer
In the vapor-solid diffusion relationship, the process of gas carburizing may be considered as diffusion. The rate-limiting phase, which kinetically becomes the controlling step of carburizing, is controlled by the gradient in the chemical potential during the process. When carbon transfer from the gas environment is equal to or higher than the rate of carbon diffusion in steel, the rate of carburization may reach its maximum. In reality, however, the process is mixed-controlled and regulated by both the mass-transfer coefficient and carbon diffusivity of steel (Wołowiec-Korecka, Citation2018; Brunatto et al., Citation2018; Wu et al., Citation2014; Eaton-Mckay et al., Citation2021). The gradient in the chemical potential of carbon drives mass transfer during carburizing according to the thermodynamics of irreversible processes (Khan & Gautham, Citation2018). By using thermodynamic carbon activity and carburizing temperature, the chemical potential of carbon was calculated using the following EquationEquation (1)(1)
(1) . where
is the carbon chemical potential, the universal gas constant is R, T is the carburizing temperature in K, and the activity of carbon is represented by
(1)
(1)
The majority of carbon activity models created and reported to date have been designed for ‘ternary Fe-C-X systems’. These hypotheses are supported by the localized forces of interactions between carbon atoms and their specific localized distribution inside the matrix of alloyed austenite (Wu et al., Citation2011; Wu et al., Citation2014). The carbon activity and carbon diffusion coefficient in austenite are increased by the addition of Ni to the steel. However, the carburizing process is hampered by the presence of alloying components in the steel. In contrast, accelerating the overall carburizing performance of steel, Cr and Mo, reduces the carbon diffusivity in austenite (Jung et al., Citation2009). The complexity of alloy steel composition leads to more complicated manifestations of these processes. Because alloy steels may be hardened by carburizing them, it is crucial to understand how alloying components affect the carburizing behavior of medium- and high-alloy steels to obtain consistent and well-controlled outcomes (Wołowiec-Korecka, Citation2018; Wu et al., Citation2014). These data and analyses are offered in a user-friendly manner by a number of thermodynamic databases, notably Thermo-Calculators (Yan et al., Citation2021).
Considering that no soot deposit occurs, based on Fick’s 2nd law, at the interface of the carburizing gas and the juncture of steel, the carburization model is given by EquationEquation (2)(2)
(2) . Based on this equation, the flow of carbon atoms diffusing into steel equals the flux of carbon from the carburizing environment to the surface.
(2)
(2)
where ki is the atmospheric chemical reaction rate coefficient, the carbon activity in the steel (surface) and carburizing gas is given by
the diffusivity of carbon in austenite is D, and the diffusion depth in the steel case is represented by x. The summation symbol (Σ) denotes the possibility of many chemical carburizing processes occurring concurrently. The coefficient of mass transfer is defined as the ratio of the coefficient of the chemical reaction rate to the activity of carbon at the surface of steel for steels with carbon content profiles of less than 1 wt.% C (Wang et al., Citation2017; Kowser & Motalleb, Citation2015).
According to previous reports, the temperature, carburizing potential, and atmospheric gas composition all influence the mass-transfer coefficient ‘β’ in various ways. As far as the authors are aware, there hasn’t been much work published that quantifies the relationship between alloy composition and the rate of carbon transfer in gas atmospheres and across gas/steel interfaces (Zhang et al., Citation2022; Wu et al., Citation2015; An et al., Citation2021; Khan et al., Citation2019). The observed differences in the effective case depth and the development of carbon concentration in different alloy steels might be explained by modelling the carbon concentration profiles using a concentration dependent ‘β’.
The crucial characteristic that is affected by the carburization temperature and steel alloy content is the diffusivity of carbon in gamma-iron (D). While this impact may not be substantial for steels with low alloy content, it may be important for steels with high alloy content and should be considered (Prasanthi et al., Citation2014; Chai et al., Citation2021). Expertise of thermodynamic statistics, such as the activity coefficient and carbon mobility in the FCC structure of alloyed austenite, is necessary to comprehend the impact of alloying on carburization efficiency. Such practical information is difficult to obtain and is not easily accessible in available research. To calculate both kinetic parameters from a simplified experiment, the coefficient of mass transfer and carbon diffusivity in this study were obtained using the direct flux integration approach (Karabelchtchikova, Citation2007).
3. Materials and method
For this investigation, four steel grades with approximately equal carbon content were chosen. These were EN3, EN35, 20MnCr5, and EN353. Where EN3 is plain carbon steel, EN35 has Ni as the major alloying element, 20MnCr5 has Cr as the major alloying element, and EN353 has both Ni and Cr. Cr is a ferrite stabilizer, and Ni is an austenite stabilizer. To determine the influence of these stabilizing elements, the aforementioned steels were selected. lists the chemical compositions of the steels. The concentrations of the principal alloying elements in different combinations were considered when choosing the steel grades (Ni, Mn, Cr, and Si). This was meant for carburizing EN3 to act as a benchmark for analyzing how the steel composition affects the carburizing performance.
Table 1. Chemical composition of the steel.
shows the microstructures of the steels under the as-received condition. The plain carbon steel had fine pearlite with a ferrite matrix, whereas the alloy steels displayed distorted pearlite in the ferrite matrix. This is due to the presence of alloying elements which have influenced the Time Temperature Transformation Curve (TTT) and resulted in distorted microstructure under continuous uncontrolled cooling (Akbarpour et al., Citation2021; Lopez-Garcia et al., Citation2022). Because there was a similar trend in the alloy steels, only one image is shown here. The samples were originally cleaned under alkaline conditions before being subjected to gas carburization. Before placing them in the carburizing furnace, all samples were first connected to metal wires and placed into fixtures (). This aids in consistent heating and carburization of all samples. Now, The furnace is prepared by purging, which involves heating it to 930 °C for approximately 30 min while reducing the amount of air it is exposed to. In this instance, 1.5 l/h of liquid propane is added through the spray. This keeps the pressure positive and prevents outside air from entering the furnace. Moreover, purging prevents steel from oxidizing. After obtaining a steady environment, the samples were placed in the furnace and sealed. As soon as the furnace reaches a temperatures of above 750 °C, liquid propane is continually sprayed into it. Boosting, Diffusion, and Equalizing are the three steps of carburizing that occur in furnaces. To speed up diffusion, samples were heated at 930 °C for 2–4 h while maintaining a carbon potential of 0.9%–1.1%. After boosting, the diffusion occurred. Again, the samples were maintained at 930 °C for 2–4 h while the carbon potential was kept between 0.8% and 0.9%. To prevent air hardening, equalizing was performed by lowering the temperature to 820 °C, where the carbon potential was maintained for 30 min at 0.6%–0.7%. Finally, each sample was cooled in a furnace to room temperature. Vent holes are used to burn unburned gases and prevent dangerous gases from entering the atmosphere. The following reactions occur during carburization (Hiremath et al., Citation2020):
Figure 2. Microstructure of steels in as received condition (a) plain carbon steel and (b) alloy steels.
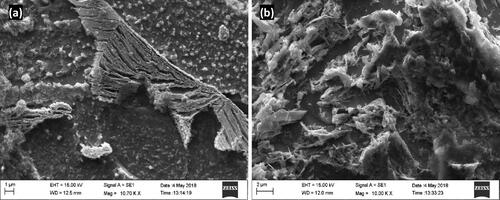
Hydrogen and carbon dioxide react with each other and increase carbon monoxide concentration by the following reaction:
Both the reaction products and
are decarburizing agents, and as their concentration increases, the carbon potential of the furnace atmosphere decreases, necessitating the addition of hydrocarbons to cause carbon enrichment on the surface.
The weight increase after carburizing was measured using a laboratory scale with a 0.1 mg precision, the weight rise after carburizing was measured. With an accuracy of 0.01 percent by weight C, the surface carbon density and carbon concentration profiles were determined using spectral analysis and a LECO optical emission spectrometer (OES). A layer of the alloy that was precisely known to be at a certain depth was gradually scraped from the surface and its chemical composition was examined. This technique was used again to detect the carbon concentration profiles until a zero-carbon gradient (for three consecutive measurements) was attained, indicating the amount of bulk carbon. Using the cubic-spline interpolation technique implemented in MATLAB, empirically obtained carbon concentration profiles were interpolated to create smooth curves for examination (Sulistiyono et al., Citation2021). A series of distinctive cubic polynomials was fitted between the data points using the cubic spline interpolation technique to ensure that the curve created was consistent and seemed seamless. The slope and integrated area beneath the curve, which are necessary for carbon diffusivity computations, were then determined using interpolated carbon concentration profiles, as discussed in the subsequent subsections.
DICTRA simulations were conducted to analyze the diffusion-controlled phase transformations in the steel alloys under investigation. The DICTRA simulations were performed using software equipped with predefined material parameters, including composition, temperature, and boundary conditions. Initially, a computational model was set up based on these parameters, representing the steel alloys and the carburization process. The simulation then employed numerical methods to calculate the diffusion of atoms over time, considering concentration gradients and diffusion coefficients within the material. As the diffusion progressed, the model tracked the phase transformations occurring in the steel alloys, such as the formation of carbides or changes in crystal structure. The output of the DICTRA simulations provided valuable insights into concentration profiles and phase fractions, which were compared with experimental results for validation purposes. This approach allowed for a comprehensive understanding of the diffusion processes occurring during carburization, aiding in the optimization of heat treatment procedures and the development of new alloy compositions.
4. Results and discussion
shows the microstructure of the steel case region after carburization. Complete pearlite transformation indicates effective carburization, as the carbon content is close to the eutectoid composition. In the presence of alloying elements, the alloy steels produced fine pearlite () compared with the unalloyed steel (). The presence of alloying elements leads to a higher degree of supercooling and results in fine pearlite (Dey et al., Citation2018). The scientifically determined carbon density profiles of the carburized components at 2 and 4 h are shown in . Despite the fact that all pieces were carburized within a similar bin in the same procedures, scientific examination showed that these steels had unique variations in their carbon concentration profiles and overall weight gains (ΔM). The Unalloyed steel was found to have the highest carbon absorption. The carbon concentration profiles in alloy steels were lower than those in plain carbon steel, based on the degree of alloying and atomic interconnections between carbon and alloying elements. The observed diffusion depth fluctuation was determined to be around 0.03 mm upon 2 h of carburization and around 0.06 mm for 4 h of carburization, if the effective case-depth is considered as the depth to 0.4 wt% C. The main variations in the concentration of carbon profiles were seen at the adjacent surface layer up to a depth of 0.4 mm, according to , after 2 h. The amount of carbon movement from the environment to the steel case region, which is kinetically the rate-limiting activity during the first stage of carburization, was ascribed to this study. The mechanism eventually shifts to a combined control, when both the coefficient of mass transfer and carbon diffusivity influence the development of the carbon profile (). In this phase, the equilibrium between the carbon flux from the gas atmosphere to the case of steel and the pace of carbon diffusion in steel determines the momentary carbon flux across the steel-gas interface (Prasanthi et al., Citation2014). Consequently, the influence of the alloying content on the carbon interaction in the steel case region and the carbon diffusion in austenite are the causes of the overall observed differences in the carburizing performance of different alloy steels.
Figure 4. Microstructure of steel’s case region after carburization (a) plain carbon steel and (b) alloy steels.
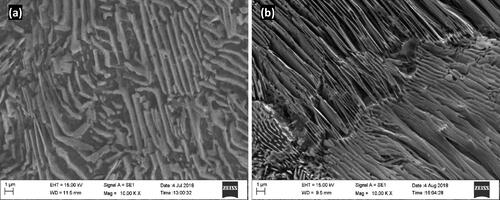
Figure 5. Concentration of carbon at different depths below the surface of the steel samples (a) 2 h of carburization and (b) 4 h of carburization.
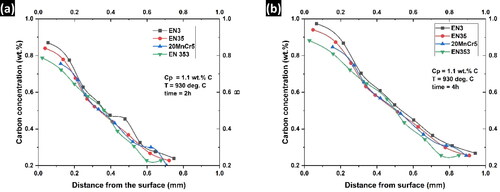
The quantity of carbon in equilibrium with the surface carbon level in unalloyed austenite is known as the carbon potential (CP) in the atmosphere. The kinetics of carbon activity in austenite and the associated coefficients for different alloyed steels were estimated using Thermo-Calculator (Ma et al., Citation2022) and are listed in for the specified carburizing temperature and CP of 1.1 wt. %. The highest equilibrium carbon concentration of the three alloy steels, 20MnCr5 steel (with high Cr and very minimal Ni contents), demonstrates the largest propensity to lower the carbon activity from its unalloyed equivalent, EN3. With a more evenly distributed ratio of austenite-stabilizing and carbide-formable alloy contents, EN353 slightly increases the equilibrium carbon concentration compared with plain carbon steels. The EN 35 steel, whose chemical content greatly lowers the equilibrium concentration of carbon in austenite and increases the carbon activity to higher temperatures in steel, has the inverse result. These findings were subsequently utilized to demonstrate how the composition of steel affects the kinetic carburizing characteristics.
Table 2. Different steels’ thermodynamic properties as estimated at 930 °C.
4.1. Coefficient of mass transfer
The actual quantity of carbon particles that were diffused into the case for a unit area may be determined from the region below the carbon density curve, the integrated carbon flow from the flux-balance status at the steel-to-gas interface, and the continuity equation of mass deposition inside steel:
(3)
(3)
where
is the flux of carbon,
is the depth from the surface after which there will not be any carbon gradient,
and
are the carburization starting and ending times, and ΔM/A is the increase in weight per unit area of the samples. The following expression (Equation (4)) for the flux of carbon at a specific time t through the gas-steel junction was obtained by further differentiating the mass gain throughout the carburization time:
(4)
(4)
The momentary coefficient of mass transfer can be used to determine the rate of carbon movement at the steel-gas junction under the assumption that the mechanism is time dependent.
(5)
(5)
or
(6)
(6)
where t is the time of carburization,
is the time at which carburization is started, and (ΔM/A)
is the increase in the weight of the carburized samples per unit area at random time instance t. The observed weight increment in the samples and the case region carbon density after 2 and 4 h of carburization are shown in . According to Equations (4) and Equation(6)
(6)
(6) , the mass transfer coefficient and carbon flow were computed from observed measurements. While it has been demonstrated that the coefficient of mass transfer (b) varies somewhat with time as the concentration of surface carbon increases, it is generally believed that β is constant for any given set of carburization circumstances (e.g. carbon potential and alloy composition) (Ernst et al., Citation2011; Wu et al., Citation2014; An et al., Citation2021). The averages of the computed values
and
are shown in .
Table 3. Coefficient of mass transfer calculations.
The computed coefficients of mass transfer varied from 8.85 × 10−6 to 1.87 × 10−5 cm/s based on the steel alloying content, while the components underwent identical carburization procedures. A minimal weight increase was observed following carburizing EN 35 steel, which also showed the weakest rates of carbon diffusion from the gas environment to the steel case. shows that the austenite stabilizer (Ni) enhance the carbon action () at the steel asec while decreasing the optimum carbon composition. As a result, the coefficient of mass transfer and the carbon flux [
penetrating the steel case are reduced. In contrast, ferrite stabilizer elements (Cr and Mo) enhance the overall carbon flow across the gas-steel contact while reducing carbon activity. The computed coefficients of mass transfer for 20MnCr5 and EN353 were better than those for EN35, revealing a greater weight increase and higher surface carbon density after carburization.
4.2. Austenitic carbon diffusivity
Similar to the computations related to mass transfer, integrating the carbon diffusion parameters across the depth until which the gradient is present, the mass gain of carbon particles seeping into the steel over an imaginary plane parallel to it can be calculated using:
(7)
(7)
Here, is the bulk concentration of carbon and
is the concentration of carbon at any depth (
). Presuming a homogenous medium, the density gradient measured transverse to the area is proportional to the flow of diffusing particles across a unit area:
(8)
(8)
The following formula for determining the carbon diffusivity from the concentration profiles can be constructed by equating EquationEquations (7)(7)
(7) and Equation(8)
(8)
(8) .
(9)
(9)
To calculate the carbon diffusivity, two factors must be combined: (i) the integrated region underneath the concentration profile differentiated with reference to carburization time and (ii) the negative inversion of the slope at any location on the carbon concentration profile. The carbon atom diffusion equation derived from the measured carbon level trends () is shown in . The estimated information was correlated to the carbon diffusivities computed from the DICTRA thermal and dynamical datasets (Prasanthi et al., Citation2014). The datasets showed a high degree of similarity.
Figure 6. Carbon diffusivities calculated and those from DICTRA are compared, (a) EN3, (b) EN35, (c) 20MnCr5 and (d) EN353.
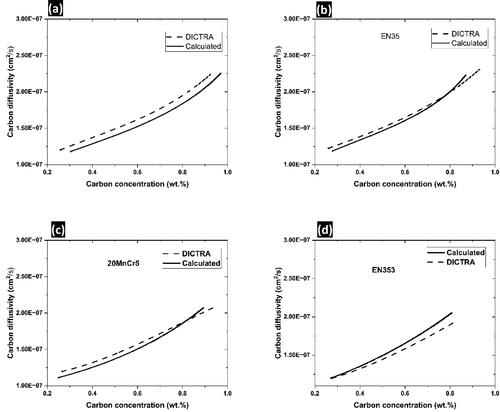
The diffusion of carbon in gamma iron for different steels is compared in . The presence of potent austenite-stabilizing elements (Ni and Si) improves austenitic carbon diffusion, notwithstanding the least coefficient mass transfer associated with EN35. This finding was ascribed to the weaker atomic interactions and lower bonding energies between the carbon atoms and austenite-stabilizing alloys. Mo and Cr, two elements that may form carbides, promote favourable atomic relations, and have a propensity to draw meticulously diffusing carbon atoms. Such outliers reduce the effective diffusivity of carbon by impeding the protracted diffusion of carbon atoms in gamma iron. The predicted carbon diffusivities of the EN353 and EN3 steels were found to be between those of EN35 and 20MnCr5 because the influence of carbide-forming alloys is countered by the presence of austenite-stabilizing elements.
Thus, this study suggests that medium- and high-alloy steels with high chromium and low nickel contents greatly decrease the ability of steel to diffuse carbon atoms, necessitating a longer carburizing time to attain the necessary diffusion depth. When such steel parts are carburized alongside high-nickel steel components, case-depth differences within the same workload are unavoidable. To reach the necessary diffusion depth, it is advised that such steel parts be carburized in a different batch and that the carburizing duration is modified correspondingly.
The significance of comprehending and calculating how the chemical content of alloy steel affects how well it carburizes should not be undervalued. The coefficient of mass transfer and diffusivity of carbon in gamma-iron changes depending on the alloy content of the steel at a certain carburization temperature. and a gaseous carburizing environment. This suggests that various alloy steels may need to be carbureted for varying lengths of time to attain an appropriate diffusion depth. A better diffusion depth consistency may be attained by including concentration-dependent b and D in the existing carburizing methods.
5. Conclusion
The study investigated the thermodynamics of mass transfer during gaseous carburization and its impact on the carburizing efficiency of various alloy steels. Through a comprehensive analysis, it provided valuable insights into how alloy composition influences the carburization process. The findings underscored the significance of considering alloy content when carburizing different steels.
A notable aspect of the research was the elucidation of specific mechanisms by which alloy elements affect mass transfer and carbon diffusion. It was observed that steels with moderate to high alloy content exhibit distinct carburizing capabilities compared to unalloyed steels. The presence of austenite-stabilizing elements such as nickel (Ni) was found to influence the rate of carburization, leading to slower dynamics and reduced end carbon content profiles. Conversely, alloying elements like chromium (Cr) and molybdenum (Mo) expedited the transfer of carbon from the gaseous environment to the steel case, despite reducing carbon diffusivity within the gamma iron matrix.
Furthermore, the comparison of carbon diffusivities calculated in this study with those obtained from DICTRA datasets revealed excellent consistency, validating the reliability of the experimental approach. These findings underscored the critical role of alloy composition in gaseous carburization processes to achieve consistent and controlled outcomes.
The study’s findings contribute to the optimization of carburizing procedures in industrial applications by providing quantitative data on the impact of alloy elements and elucidating the mechanisms underlying the carburization process. By presenting clear arguments and comprehensive summaries of the research achievements, this work enhances understanding of the relevance and significance of the results. Future research endeavors could delve deeper into the interaction between alloying elements and carburization kinetics to develop more refined carburizing strategies tailored to specific alloy compositions.
Author contributions
Conceptualization: Pavan Hiremath; methodology: Pavan Hiremath, Gurumurthy B M, Shivaprakash Y M; validation: Nithesh Naik; investigation: Prateek Jain; resources: Suhas Kowshik, Prateek Jain, B R N Murthy; writing—original draft preparation: Pavan Hiremath, Nithesh Naik; writing—review and editing: Gurumurthy B M, Suhas Kowshik; visualization: Shivaprakash Y M; supervision: Pavan Hiremath, Nithesh Naik, Prateek Jain; project administration: Pavan Hiremath, Prateek Jain. All authors have read and agreed to the published version of the manuscript.
Disclosure statement
No potential conflict of interest was reported by the authors.
Data availability statement
All the data used in the experiment have been made available in the present article. The data that support the findings of this study are available from the corresponding author, [email protected] or COGENT Engineering, upon reasonable request.
Additional information
Funding
Notes on contributors
Pavan Hiremath
Dr. Pavan Hiremath holds M.Tech in Manufacturing Technology, and a PhD in Material Science and Engineering with a focus on Heat Treatment from Manipal Academy of Higher Education, Manipal. He began his career at the National Aerospace Laboratories in Bangalore, contributing significantly to the SARAS aircraft project, India’s first multi-purpose civilian aircraft in the light transport category. Dr. Hiremath is now a Associate Professor in the Department of Mechanical and Industrial Engineering at the Manipal Institute of Technology (MIT), where he mentors Ph.D. students and oversees various undergraduate and postgraduate projects. His academic journey is marked by a commitment to rigorous research, evidenced by over 70 publications in prestigious international journals and conferences. Dr. Hiremath is also a member of MAHE’s Strategy and Innovation Group, contributing to the institution’s vision and fostering innovation in academia. He worked on six patents, including the “X-ray chair” for accurate medical imaging, innovations in precipitation hardening of aluminum alloys for lightweight yet high-strength materials, and capacitive sensors for optimizing agricultural processes.
Gurumurthy B. M.
Dr. Gurumurty B.M. is an Associate Professor in the department of Mechanical and Industrial Engineering, Manipal Institute of Technology, Manipal, India. He is a Mechanical Engineer with a master’s in manufacturing engineering and technology and Ph. D in Heat treatment and Machining Materials. He has more than 13 years in teaching, industry, and research experience. His areas of interest include Heat treatment, characterization of composites, machining. He has published 50 (Scopus indexed) research papers in reputed international journals and presented 10 research papers in international conferences.
Shivaprakash Y. M.
Dr. Shivaprakash Y.M. is an Additional Professor in the department of Mechanical and Industrial Engineering, Manipal Institute of Technology, Manipal, India. He is a Mechanical Engineer with a master’s in manufacturing engineering and technology and Ph. D in Composite Materials. He has more than 18 years in teaching, industry, and research experience. His areas of interest include Heat treatment, characterization of composites and hybrid composites. He has published 25 (Scopus indexed) research papers in reputed international journals and presented 12 research papers in international conferences.
Nithesh Naik
Prof. Nithesh Naik is a Senior Assistant Professor in the department of Mechanical and Industrial Engineering, Manipal Institute of Technology, Manipal, India. He is a Mechanical Engineer with a Masters in Computer Aided Machine Design and is presently pursuing Ph.D in composite Materials. He has more than 10 years in teaching, industry and research experience. His areas of interest include characterization of polymer matrix composites, bio composites and hybrid composites. He has published 170 (Scopus indexed) research papers in reputed international journals and presented 15 research papers in international conferences.
Prateek Jain
Dr. Prateek Jain earned his B.Tech. in Electrical Engineering from BIT, Durg, India, in 2008, his M.Tech. in Power Systems from VJTI, Mumbai, India, in 2010, and his PhD in Electrical Engineering from IIT Indore, India, in 2018. He served as a Lecturer in the Department of Electrical Engineering at VJTI, Mumbai, from 2010 to 2012. Currently, he is an Assistant Professor in the Department of Electrical and Electronics Engineering at Manipal Institute of Technology, Manipal, India, having joined the institute in 2019. Additionally, he worked as a Post-Doctoral Fellow at the Centre of Energy Regulation, Department of Industrial & Management Engineering, IIT Kanpur, India, from 2018 to 2019, where he contributed to regulatory research and energy analytics for the electricity markets in the power sector. His research interests lie in the power and energy engineering domain, focusing on electric vehicle-power grid interactions, machine learning and data science applications in energy analytics, power grid ancillary services, and smart grid.
Suhas Kowshik
Prof. Suhas Kowshik is a Senior Assistant Professor in the department of Mechanical and Industrial Engineering, Manipal Institute of Technology, Manipal, India. He is a Mechanical Engineer with a Masters in Manufacturing Engineering and Technology and is presently pursuing Ph.D in composite Materials. He has more than 10 years in teaching, industry and research experience. His areas of interest include characterization of polymer matrix composites, bio composites and hybrid composites. He has published 24 (Scopus indexed) research papers in reputed international journals and presented 10 research papers in international conferences.
Murthy B. R. N.
Dr. Murthy B. R. N. is currently working as Additional Professor in the Department of Mechanical and Industrial Engineering, Manipal Institute of Technology, Manipal Academy of Higher Education, Manipal, India. He is the author for several articles in various International Journals. His research includes optimization and simulation of machining process on various types of fiber re-in forced polymer composites through Taguchi, Response Surface Methodology, Artificial Neural Networks and System Dynamic Methods. His field of research also includes conduction of Austempering heat treatment to improve the performance of Austempered Ductile Iron Balls which are recognized as the better grinding media for grinding iron ores in ball mills.
References
- Abdulrazzaq Jabbar, D., & Kadhim, Z. D. (2020). Investigation and characterization of coating and carburizing AISI 1011 steel. IOP Conference Series: Materials Science and Engineering, 765(1), 12065. https://doi.org/10.1088/1757-899X/765/1/012065
- Akbarpour, M. R., Mashhuriazar, A., & Daryani, M. (2021). Experimental and numerical investigation on the effect of the tempcore process parameters on microstructural evolution and mechanical properties of dual-phase steel reinforcing rebars. Metals and Materials International, 27(10), 4074–4083. Oct https://doi.org/10.1007/s12540-020-00840-4
- An, X., Tian, Y., Wang, B., Jia, T., Wang, H., & Wang, Z. (2021). Prediction of the formation of carbide network on grain boundaries in carburizing of 18CrNiMo7-6 steel alloys. Surface Coatings Technology, 421, 127348. https://doi.org/10.1016/j.surfcoat.2021.127348
- Boubaker, K. M., Bouhafs, M., & Yacoubi, N. (2003). A quantitative alternative to the Vickers hardness test based on a correlation between thermal diffusivity and hardness—Applications to laser-hardened carburized steel. NDT and E International, 36(8), 547–552. https://doi.org/10.1016/S0963-8695(03)00053-7
- Brunatto, S. F., Scheuer, C. J., Boromei, I., Martini, C., Ceschini, L., & Cardoso, R. P. (2018). Martensite coarsening in low-temperature plasma carburizing. Surface Coatings Technology, 350, 161–171. https://doi.org/10.1016/j.surfcoat.2018.07.002
- Chai, J., Pestman, R., Chen, W., Dugulan, A. I., Feng, B., Men, Z., Wang, P., & Hensen, E. J. M. (2021). The role of H2 in Fe carburization by CO in Fischer-Tropsch catalysts. Journal of Catalysis, 400, 93–102. https://doi.org/10.1016/j.jcat.2021.05.027
- Dey, I., Chandra, S., Saha, R., & Ghosh, S. K. (2018). Effect of Nb micro-alloying on microstructure and properties of thermo-mechanically processed high carbon pearlitic steel. Materials Characterization, 140, 45–54. https://doi.org/10.1016/j.matchar.2018.03.038
- Eaton-Mckay, J., Yan, K., Zhong, X., Callaghan, M. D., & Jimenez-Melero, E. (2021). Oxidation and carburization behaviour of two type 316H stainless steel casts in simulated AGR gas environment at 550 and 600 °C. Journal of Nuclear Materials, 552, 152999. https://doi.org/10.1016/j.jnucmat.2021.152999
- Egawa, M., Ueda, N., Nakata, K., Tsujikawa, M., & Tanaka, M. (2010). Effect of additive alloying element on plasma nitriding and carburizing behavior for austenitic stainless steels. Surf. Coatings Technol, 205, S246–S251. https://doi.org/10.1016/j.surfcoat.2010.07.093
- Ernst, F., Li, D., Kahn, H., Michal, G. M., & Heuer, A. H. (2011). The carbide M7C3 in low-temperature-carburized austenitic stainless steel. Acta Materialia. 59(6), 2268–2276. https://doi.org/10.1016/j.actamat.2010.11.058
- Gu, X., Michal, G. M., Ernst, F., Kahn, H., & Heuer, A. H. (2014). Concentration-dependent carbon diffusivity in austenite. Metallurgical and Materials Transactions A, 45(9), 3790–3799. https://doi.org/10.1007/s11661-014-2347-5
- Heintzberger, P. J. (2020). Influence of the temperature of vacuum carburizing on the thickness of the carburized layer and properties of steel parts. Metal Science and Heat Treatment, 62(3-4), 279–284. https://doi.org/10.1007/s11041-020-00549-6
- Hiremath, P., Sharma, S., M.c, G., Shettar, M., & B.m, G. (2020). Effect of post carburizing treatments on residual stress distribution in plain carbon and alloy steels – A numerical analysis. Journal of Materials Research and Technology, 9(4), 8439–8450. https://doi.org/10.1016/j.jmrt.2020.05.104
- Jiang, Y., Wu, Q., Wang, Y., Zhao, J., & Gong, J. (2019). Suppression of hydrogen absorption into 304L austenitic stainless steel by surface low temperature gas carburizing treatment. International Journal of Hydrogen Energy, 44(43), 24054–24064. https://doi.org/10.1016/j.ijhydene.2019.07.112
- Jung, M., Oh, S., & Lee, Y.-K. (2009). Predictive model for the carbon concentration profile of vacuum carburized steels with acetylene. Metals and Materials International, 15(6), 971–975. https://doi.org/10.1007/s12540-009-0971-1
- Karabelchtchikova, O. (2007). Fundamentals of mass transfer in gas carburizing. [Doctoral Dissertation Submitted to the Faculty of the Worcester Polytechnic Institute]. https://vdocuments.net/gas-carburizing.html?page=1.
- Khan, D., & Gautham, B. (2018). Integrated modeling of carburizing-quenching-tempering of steel gears for an ICME framework. Integrating Materials and Manufacturing Innovation, 7(1), 28–41. Mar https://doi.org/10.1007/s40192-018-0107-x
- Khan, D., Shukla, R., & Gautham, B. P. (2019). In silico design of materials and processes: An application of ICME to carburizing steels. Transactions of the Indian Institute of Metals, 72(8), 2179–2185. Aug https://doi.org/10.1007/s12666-018-1534-2
- Kim, D.-W., Cho, Y.-G., Cho, H.-H., Kim, S.-H., Lee, W.-B., Lee, M.-G., & Han, H. N. (2011). A numerical model for vacuum carburization of an automotive gear ring. Metals and Materials International, 17(6), 885–890. https://doi.org/10.1007/s12540-011-6004-x
- Kowser, M. A., & Motalleb, M. A. (2015). Effect of quenching medium on hardness of carburized low carbon steel for manufacturing of spindle used in spinning mill. Procedia Engineering. 105, 814–820. https://doi.org/10.1016/j.proeng.2015.05.076
- Lopez-Garcia, R. D., Medina-Juárez, I., & Maldonado-Reyes, A. (2022). Effect of quenching parameters on distortion phenomena in AISI 4340 steel. Metals (Basel, 12(5), 759. https://doi.org/10.3390/met12050759
- Ma, W., Sheng, J., Wang, Y., Yan, M., Wu, Y., Qin, S., Zhou, X., & Zhang, Y. (2022). Measurements of Carbon Diffusivity and Surface Transfer Coefficient by Electrical Conductivity Relaxation during Carburization: Experimental Design by Theoretical Analysis. Coatings, 12(12), 1886. https://doi.org/10.3390/coatings12121886
- Ochsner, A., Gegner, J., & Mishuris, G. (2004). Effect of diffusivity as a function of the method of computation of carbon concentration profiles in steel. Metal Science and Heat Treatment. 46(3/4), 148–151. https://doi.org/10.1023/B:MSAT.0000036667.33197.00
- Prasanthi, T. N., Sudha, C., Paul, V. T., Bharasi, N. S., Saroja, S., & Vijayalakshmi, M. (2014). Modification in the microstructure of mod. 9Cr-1Mo Ferritic martensitic steel exposed to sodium. Metallurgical and Materials Transactions A, 45(10), 4220–4234. https://doi.org/10.1007/s11661-014-2361-7
- Rowan, O. K., & Sisson, R. D. (2009). Effect of alloy composition on carburizing performance of steel. Journal of Phase Equilibria and Diffusion, 30(3), 235–241. https://doi.org/10.1007/s11669-009-9500-7
- Sharghi-Moshtaghin, R., Kahn, H., Ge, Y., Gu, X., Martin, F. J., Natishan, P. M., Rayne, R. J., Michal, G. M., Ernst, F., & Heuer, A. H. (2010). Low-temperature carburization of the Ni-base superalloy IN718: Improvements in surface hardness and crevice corrosion resistance. Metallurgical and Materials Transactions A, 41(8), 2022–2032. https://doi.org/10.1007/s11661-010-0299-y
- Sulistiyono, B., Irawan, Y. S., Suprapto, A., & Soenoko, R. (2021). The comparison pack carburizing-nitriding SUS 316 with gas type welding grade and ultra high purity. EUREKA: Physics and Engineering, 3, 119–126. https://doi.org/10.21303/2461-4262.2021.001839
- Trotea, M., Constantinescu, A., Simniceanu, L., & Pană, G. M. (2018). Influence of carburizing time of iron powder samples on the carbon content after gas-carburizing – Sintering process. Applied Mechanics and Materials, 880, 235–240. www.scientific.net/AMM.880.235. https://doi.org/10.4028/www.scientific.net/AMM.880.235
- Wang, J., Li, Z., Wang, D., Qiu, S., & Ernst, F. (2017). Thermal stability of low-temperature-carburized austenitic stainless steel. Acta Materialia. 128, 235–240. https://doi.org/10.1016/j.actamat.2017.02.018
- Wei, S., Wang, G., Zhao, X., Zhang, X., & Rong, Y. (2014). Experimental study on vacuum carburizing process for low-carbon alloy steel. Journal of Materials Engineering and Performance, 23(2), 545–550. Feb https://doi.org/10.1007/s11665-013-0762-1
- Wołowiec-Korecka, E. (2018). Modeling methods for gas quenching, low-pressure carburizing and low-pressure nitriding. Engineering Structures, 177, 489–505. https://doi.org/10.1016/j.engstruct.2018.10.003
- Wu, D., Ge, Y., Kahn, H., Ernst, F., & Heuer, A. H. (2015). Diffusion profiles after nitrocarburizing austenitic stainless steel. Surface Coatings Technology, 279, 180–185. https://doi.org/10.1016/j.surfcoat.2015.08.048
- Wu, J., Xue, W., Wang, B., Jin, X., Du, J., & Li, Y. (2014). Characterization of carburized layer on T8 steel fabricated by cathodic plasma electrolysis. Surface Coatings Technology, 245, 9–15. https://doi.org/10.1016/j.surfcoat.2014.02.024
- Wu, Y., Duan, G. S., Lu, Y., Zhao, X., & Zuo, L. (2011). Effects of magnetic field annealing on carburizing in pure iron. Steel Research International, 82(12), 1404–1407. https://doi.org/10.1002/srin.201100117
- Yan, F., Yao, J., Chen, B., Yang, Y., Xu, Y., Yan, M., & Zhang, Y. (2021). A novel decarburizing-nitriding treatment of carburized/through-hardened bearing steel towards enhanced nitriding kinetics and microstructure refinement. Coatings, 11(2), 112. https://doi.org/10.3390/coatings11020112
- Zhang, N., Guo, S., He, G., Jiang, B., Zhou, L., Chen, Y., & Liu, Y. (2022). Failure analysis of the carburized 20MnCr5 gear in fatigue working condition. International Journal of Fatigue, 161, 106938. https://doi.org/10.1016/j.ijfatigue.2022.106938